The industry gathered at the majestic Palau National to celebrate the SBC Awards 2022 winners
September 23, 2022
Katerina Biloruska, Parimatch Tech: Ukraine is in our DNA
September 23, 2022
Platelets in Wound Resolution
Platelets release significant amounts of factors from α-granules such as α2-macroglobulin, plasminogen activator, plasminogen, and plasminogen-activator inhibitor type-1 that help with fibrinolysis, clot dissolution, inflammation resolution, and wound repair (113–117). They also release tissue remodeling enzymes involved in wound repair that include matrix metalloproteinases (MMP-1, MMP-2, MMP-3, MMP-9, MT1-MMP, MMP-14), tissue inhibitor of metalloproteinases (TIMPs; TIMP-1, TIMP-2 and TIMP-4), as well as a disintegrin and metalloproteinases (ADAMs; ADAM-10, ADAM-17, ADAMTS-13) (118, 119). Platelet-activating factor induces the expression of a number of tissue-remodeling proteases (120–122). PDGF also stimulates tissue remodeling hepatic stellate cell (HSC) proliferation and fibrosis in the liver that involves tissue-remodeling proteases (123). MMPs secreted by activated platelets play an integral role in tumor spread and the metastatic cascade by contributing in tissue remodeling and stimulating tumor-cell transmigration and invasion of surrounding tissues, blood vessels, and liver sinusoids (124–128).
Platelets as First Responders
Platelets can be considered first responders in the context of wounding, tissue repair, and inflammatory and immune responses that occur with cancer progression and metastasis. Platelets are important anucleate elements of the immune and hemostatic systems. Their genesis occurs when membrane-bound organelles containing cytoplasmic extrusion are released as small (2-3 μm) blebs into the blood stream. This blebbing, subcellular, genesis process occurs from surfaces of megakaryocytes, the largest cells in the body, and occurs primarily in the bone marrow. Normal human platelets are principally considered to be responsible for coagulation and fibrinolysis, but emerging data suggest a greater impact on immunology and cancer biology.
Resting platelets are plate-like discs that maximize planar surface interactions (9–11) that are biophysically concentrated toward the outer fluid-shear fields of flowing blood—much like silt movements associated with wave action (12–19). The liver sinusoids exhibit their own unique uneven wall/fluid-shear characteristics due to the open irregular sinusoidal vasculature compared to the typical vessels that are fully enveloped by a smooth continuous layer of endothelial cells and are subject to more uniform laminar field shear (20–23). These liver-sinusoidal vessel properties can change with liver fibrosis, metastasis, and damage from chemotherapy (24–28). Taken together, these properties enhance encounters with and recognition of any vascular wall lesions, wounds, or tumors. Should platelets encounter basement membrane or underlying matrix, they undergo receptor-mediated activation (29–34) connected to very rapid cytoskeleton- and membrane changes to form filopodia leading to adhesion (35–42). This rapid process occurs within seconds along with shape change and exocytic degranulation. In turn, degranulation encompasses the release of proteins, growth factors, cytokines, and lysozymes accompanied by a variety of bioactive lipids, small molecules, and other factors. Successful sequential recruitment of additional platelets and immune cells coupled with thrombogenesis ultimately seals any tissue gaps and initiates recruitment of other immune cells.
Liver Damage or Injury and Coagulation
Liver disease can manifest through several mechanisms. Overuse of certain drugs or alcohol, metabolic syndrome, diabetes, chronic viral infection, and exposure to toxins are some of the many contributors to liver injury and disease. Depending on the degree of liver damage, individuals with liver disease have deficiencies in clotting enzymes, which manifest as prolonged clotting times in in vitro assays. Chronic liver-disease-associated coagulation disorders result from the inability of the liver to produce or clear clots (194, 199). Imbalances in the synthesis or clearance of clotting factors can increase the risk of bleeding; increasing evidence suggests that these also increase the risk of prothrombotic events (156). The development of coagulopathies is associated with chronic liver disease; circulating levels of some coagulation factors such as vWF and factors II, -V, and -VII have been shown to correlate with the severity of liver disease. Blood coagulation-protein levels can also reflect liver-cell functionality (200–202). In some instances, irregularities in these levels can contribute to the process of liver damage.
Platelet Clearance
The body is thought to produce and clear 1011 platelets per day (135, 136); clearance occurs primarily in the liver and spleen. Clearance mechanisms include senescence and apoptosis driven by Bcl-xL and the proapoptotic molecules Bax and Bak, which set the internal clock for platelet lifespan in conjunction with BH3-only proteins, mitochondrial permeabilization, phosphatidylserine exposure, and lectin-mediated recognition of platelet glycans (135–137). The vWF or antibodies binding to platelet surface glycoproteins under fluid shear induce mechanosensory signaling that leads to ADAM17 and phosphatidylserine exposure along with desialylation (138). These molecular changes are also commonly associated with platelet aging-related clearance and thrombocytopenia found in type 2B von Willebrand disease (139). Recognition of platelet glycans by the Ashwell-Morell receptor leads to clearance of senescent platelets by hepatocytes, macrophages, and other resident liver- or spleen immune cells. The complexities and temporal state of the platelet life-span clock and surface recognition by resident liver-immune cells is likely to influence the dynamics of liver diseases by selectively eliminating aging platelets and allowing fully functional platelets to exert their normal biological function.
Sterile Injury
Two types of liver injury—sterile and non-sterile—are mediated by similar but distinctly different platelet engaging immune responses. In the case of sterile injury, an inflammatory reaction occurs in the absence of infection during normal sterile-wound healing (7). When this process becomes dysregulated in various acute- and chronic inflammatory liver diseases (such as non-alcoholic fatty-liver disease [NAFLD]/NASH), toxic injury-altered immune-cell trafficking and abnormal function further promote progressive, chronic inflammatory disease (7). Platelets initiate the early biological responses to injury through direct contact and release of granules (173). In one sterile-injury-and-repair model of the liver, platelets were the first responders directly observed to form a substratum that facilitated neutrophil entry to the injured site for subsequent repair (8). In the sterile-inflammation case of liver ischemia/reperfusion injury, the release of damage-associated molecules can also trigger toll-like receptor 4 (TLR4)- and TLR9-MyD88 signaling pathways to form neutrophil extracellular traps (NETs) that exacerbate organ damage and initiate inflammatory responses (203). Following sterile injury to the liver, local cytokines can also reprogram classic, proinflammatory (CCR2hiCX3CR1low) monocytes into nonclassical-, patrolling-, or alternative (CCR2lowCX3CR1hi) monocytes to facilitate proper wound-healing (204).
Liver Coagulation-Factor Biology
Along with regulating platelet number, the liver also plays an important role in coagulation. Both coagulation and anti-coagulant proteins are primarily made in the liver; thus, any liver disease can potentially dysregulate coagulation (156, 194–196). Most coagulation factors are synthesized by the parenchymal cells of the liver (factors I, -II, -V, -VII, -IX, -X; proteins C, -S, and –Z; fibrinogen; antithrombin; α2-PI; and plasminogen); while factor VIII is produced by liver LSECs (197). Of these, the synthesis of factors II, -VII, -IX, -X; and proteins C and -S are dependent on vitamin K—an important cofactor for regulating coagulation. As the primary storage site for vitamin K, the liver provides conversion of synthetic vitamin K to its active form and produces the bile salts that aid with the absorption of food-based vitamin K (156, 194–196, 198). The liver also plays a role in the clearance of the coagulation products from the bloodstream and regulates anticoagulation by removing activated clotting- and fibrinolytic factors via the hepatic reticuloendothelial system.
Platelet-to-Lymphocyte Ratios
Elevations in circulating platelet-to-lymphocyte ratios are often associated with inflammation and poor outcomes along with being linked to infections, inflammatory diseases, liver disease and cancer (140–146). The predictive value indicative of poor outcomes linked to elevated platelet-to-lymphocyte ratios has shown clear associations with liver transplantation, particularly when performed for hepatocellular carcinoma and liver metastasis (147–152).
Evidence exists in support of the predictive value of platelet-to-lymphocyte ratios as a general biomarker of inflammation but prospective case-control studies in each disease will help to further establish this hypothesis.
Abbreviations
CTCs, Circulating tumor cells; CRC, colorectal cancer; EGF, epidermal growth factor; HSCs, hepatic stellate cells; LSECs, liver sinusoidal endothelial cells; MRD, minimal residual disease; NAFLD, non-alcoholic fatty liver disease; NASH, non-alcoholic steatohepatitis; PDGF, platelet-derived growth factor; TPO, thrombopoietin; TLRs, toll-like receptors; TCIPA, tumor-cell-induced platelet aggregation; VEGF, vascular endothelial growth factor; vWF, vonWillebrand factor.
Granule Release as Essential Components of the Immune System
Although platelets are principally considered to be responsible for coagulation and fibrinolysis, data suggest that platelets also serve as key effectors in immunological responses. Platelet function in normal wound biology contributes to potential pathogen clearance, tissue repair, and resolution of inflammation. Once a clot is formed, pro-inflammatory mediators attract immune cells to sterilize the resulting wound. These factors include C-X-C motif chemokines such as CXCL1 (GRO-α), CXCL4 (PF4), CXCL5 (ENA-78), CXCL7 (PBP, β-TG, CTAP-III, NAP-2), CXCL8 (IL-8), CXCL12, and stromal-cell-derived factor-α (101). Platelet-derived CXCL12 is involved in mediating inflammation, immune response resolution, and repair mechanisms within sites of tissue injury (102). CXCL12 also binds CXCR4 and CXCR7 and regulates monocyte/macrophage functions (103). CXCL4 and CXCL7 are the most abundant α-granule proteins and—following CXCL12 binding along with chemokine C-X-C-motif ligand 11 (CXCL11) and macrophage migration-inhibitory factor—help to dynamically modulate wound site biology (104, 105). In one study, when platelets were co-cultured with monocytes, they predominantly differentiated into CD163+ macrophages (103, 106), which may involve EP4-receptor stimulation (107). In cardiovascular disease, CD163+ elevation elicits the differentiation of a distinct, alternative, non–foam-cell anti-inflammatory macrophage that, in turn, promotes angiogenesis, leakiness, inflammation, and plaque progression via the CD163/HIF1α/VEGF-A pathway (108). CD163 has been used as a biomarker of the anti-inflammatory M2 macrophage phenotype in tumor-associated macrophages and has been associated with tumor progression in a number of cancers including colorectal cancer (CRC) (109). Platelet α-granules also release additional C-C motif chemokines that include: CCL2 (MCP-1), CCL3 (MIP-1α), and CCL5 (RANTES), CCL7 (MCP-3); and CCL17 (TARC) along with IL1-β, PAF acetyl hydrolase, and LPA (83). Other immuno-active molecules include C1 inhibitor and immunoglobulin-G; while other hemostasis-related α-granule proteins include albumin, α1–antitrypsin, high-molecular-weight kininogen and Gas6 (92, 93).
Degranulation and surface interactions of platelets with immune cells induce biological responses by leucocytes and progenitor- and endothelial cells at the site of pathogen permeation or vascular injury inflow. Platelet interactions with neutrophils, monocytes, and lymphocytes activate and promote platelet-leukocyte aggregates that immobilize and eliminate pathogens from spreading further. Platelets can also phagocytize pathogens directly. Platelet toll-like receptors (TLRs) also recognize and respond to pathogens in the gut microbiome (110–112).
Conclusion
Platelets are immediate responders to an injury and contribute to the coagulation process by physically plugging the wound and releasing factors that contribute in the repair process. In addition to platelets, the liver also plays a critical role in hemostasis by synthesizing coagulation pathway proteins and promoting platelet production. In conditions where the liver has sustained significant damage, coagulation- and thrombogenesis processes can become dysregulated. When combined with liver disease, thrombocytosis and platelet activation is further promoted creating a microenvironment similar to that of a wound. Activated platelet-surface interactions and granule release initiate signal transduction and biologic responses.
Analogous mechanisms come into play in the case of cancer. Platelet activation, circulating cancer exosomes, thrombosis, TCIPA, and heterotypic aggregate formation are commonly associated with cancer. In patients with cancer and liver disease the interactions between CTCs and activated platelet aggregates can increase, and platelet-tumor thrombi can get trapped within the hypercoagulative liver vasculature. The administration of chemotherapy can further worsen the hemostatic regulation in these patients. The increased formation of Trousseau’s-Syndrome-related thrombi and the increased interaction of platelets together with tumor cells create a suitable environment for tumor-cell entrapment. Given the complex, multi-faceted role of the platelet in both wound healing and cancer biology, it remains uncertain if certain cancer patients could benefit from platelet-inhibition agents not yet identified.
In conclusion, heightened liver injury may increase the retention of CTCs that triggers rapidly responding platelets and fibrinogenesis and associated immune cells at metastatic foci within liver sinusoids. In patients who have a pre-existing liver disease or who develop chemotherapy-induced liver injury, these CTCs are likely to find a supportive niche within the liver sinusoids and adjacent space of Disse that promotes liver metastasis and MRD.
References
1. Garmo C, Burns B. Physiology, Clotting Mechanism. Treasure Island (FL: StatPearls, StatPearls Publishing StatPearls Publishing LLC. (2019).
Google Scholar
2. Opneja A, Kapoor S, Stavrou EX. Contribution of Platelets, the Coagulation and Fibrinolytic Systems to Cutaneous Wound Healing. Thromb Res (2019) 179:56–63. doi: 10.1016/j.thromres.2019.05.001
PubMed Abstract | CrossRef Full Text | Google Scholar
3. Dvorak HF. Tumors: Wounds That do Not Heal. Similarities Between Tumor Stroma Generation and Wound Healing. N Engl J Med (1986) 315:1650–9. doi: 10.1056/NEJM198612253152606
PubMed Abstract | CrossRef Full Text | Google Scholar
4. Lam M, Roszik J, Kanikarla-Marie P, Davis JS, Morris J, Kopetz S, et al. The Potential Role of Platelets in the Consensus Molecular Subtypes of Colorectal Cancer. Cancer Metastasis Rev (2017) 36:273–88. doi: 10.1007/s10555-017-9678-9
PubMed Abstract | CrossRef Full Text | Google Scholar
5. Wada T, Tsuneki H, Sasaoka T. Role of Angiogenesis and Chronic Inflammation in Fat Hypertrophy in NASH Pathology. Yakugaku Zasshi (2019) 139:1163–7. doi: 10.1248/yakushi.19-00011-3
PubMed Abstract | CrossRef Full Text | Google Scholar
6. Aizarani N, Saviano A, Sagar , Mailly L, Durand S, Herman JS, et al. A Human Liver Cell Atlas Reveals Heterogeneity and Epithelial Progenitors. Nature (2019) 572:199–204. doi: 10.1038/s41586-019-1373-2
PubMed Abstract | CrossRef Full Text | Google Scholar
7. McDonald B, Kubes P. Innate Immune Cell Trafficking and Function During Sterile Inflammation of the Liver. Gastroenterology (2016) 151:1087–95. doi: 10.1053/j.gastro.2016.09.048
PubMed Abstract | CrossRef Full Text | Google Scholar
8. Slaba I, Wang J, Kolaczkowska E, McDonald B, Lee WY, Kubes P. Imaging the Dynamic Platelet-Neutrophil Response in Sterile Liver Injury and Repair in Mice. Hepatology (2015) 62:1593–605. doi: 10.1002/hep.28003
PubMed Abstract | CrossRef Full Text | Google Scholar
9. O’Brien S, Kent NJ, Lucitt M, Ricco AJ, McAtamney C, Kenny D, et al. Effective Hydrodynamic Shaping of Sample Streams in a Microfluidic Parallel-Plate Flow-Assay Device: Matching Whole Blood Dynamic Viscosity. IEEE Trans BioMed Eng (2012) 59:374–82. doi: 10.1109/TBME.2011.2172607
PubMed Abstract | CrossRef Full Text | Google Scholar
10. Jen CJ, Tai YW. Morphological Study of Platelet Adhesion Dynamics Under Whole Blood Flow Conditions. Platelets (1992) 3:145–53. doi: 10.3109/09537109209013175
PubMed Abstract | CrossRef Full Text | Google Scholar
11. Folie BJ, McIntire LV. Mathematical Analysis of Mural Thrombogenesis. Concentration Profiles of Platelet-Activating Agents and Effects of Viscous Shear Flow. Biophys J (1989) 56:1121–41. doi: 10.1016/S0006-3495(89)82760-2
PubMed Abstract | CrossRef Full Text | Google Scholar
12. Fedosov DA, Noguchi H, Gompper G. Multiscale Modeling of Blood Flow: From Single Cells to Blood Rheology. Biomech Model Mechanobiol (2014) 13:239–58. doi: 10.1007/s10237-013-0497-9
PubMed Abstract | CrossRef Full Text | Google Scholar
13. Kumar A, Graham MD. Mechanism of Margination in Confined Flows of Blood and Other Multicomponent Suspensions. Phys Rev Lett (2012) 109:108102. doi: 10.1103/PhysRevLett.109.108102
PubMed Abstract | CrossRef Full Text | Google Scholar
14. Tokarev AA, Butylin AA, Ataullakhanov FI. Platelet Adhesion From Shear Blood Flow is Controlled by Near-Wall Rebounding Collisions With Erythrocytes. Biophys J (2011) 100:799–808. doi: 10.1016/j.bpj.2010.12.3740
PubMed Abstract | CrossRef Full Text | Google Scholar
15. Tokarev AA, Butylin AA, Ermakova EA, Shnol EE, Panasenko GP, Ataullakhanov FI. Finite Platelet Size Could be Responsible for Platelet Margination Effect. Biophys J (2011) 101:1835–43. doi: 10.1016/j.bpj.2011.08.031
PubMed Abstract | CrossRef Full Text | Google Scholar
16. Lee SY, Ferrari M, Decuzzi P. Design of Bio-Mimetic Particles With Enhanced Vascular Interaction. J Biomech (2009) 42:1885–90. doi: 10.1016/j.jbiomech.2009.05.012
PubMed Abstract | CrossRef Full Text | Google Scholar
17. Stukelj R, Schara K, Bedina-Zavec A, Sustar V, Pajnic M, Paden L, et al. Effect of Shear Stress in the Flow Through the Sampling Needle on Concentration of Nanovesicles Isolated From Blood. Eur J Pharm Sci (2017) 98:17–29. doi: 10.1016/j.ejps.2016.10.007
PubMed Abstract | CrossRef Full Text | Google Scholar
18. De Gruttola S, Boomsma K, Poulikakos D. Computational Simulation of a non-Newtonian Model of the Blood Separation Process. Artif Organs (2005) 29:949–59. doi: 10.1111/j.1525-1594.2005.00164.x
PubMed Abstract | CrossRef Full Text | Google Scholar
19. Nesbitt WS, Westein E, Tovar-Lopez FJ, Tolouei E, Mitchell A, Fu J, et al. A Shear Gradient-Dependent Platelet Aggregation Mechanism Drives Thrombus Formation. Nat Med (2009) 15:665–73. doi: 10.1038/nm.1955
PubMed Abstract | CrossRef Full Text | Google Scholar
20. Ogiso S, Nakamura M, Tanaka T, Komiya K, Kamei H, Onishi Y, et al. Computational Fluid Dynamics-Based Blood Flow Assessment Facilitates Optimal Management of Portal Vein Stenosis After Liver Transplantation. J Gastrointest Surg (2019) 24:460–1. doi: 10.1007/s11605-019-04279-w
PubMed Abstract | CrossRef Full Text | Google Scholar
21. Liu J, Tian L, Wang S, Luo Z. Study of Hepatic Vascular Dynamics Based on Symmetrical Pulsating Perfusion. Ann Transplant (2019) 24:214–22. doi: 10.12659/AOT.913008
PubMed Abstract | CrossRef Full Text | Google Scholar
22. Pasalic L, Wing-Lun E, Lau JK, Campbell H, Pennings GJ, Lau E, et al. Novel Assay Demonstrates That Coronary Artery Disease Patients Have Heightened Procoagulant Platelet Response. J Thromb Haemost (2018) 16:1198–210. doi: 10.1111/jth.14008
PubMed Abstract | CrossRef Full Text | Google Scholar
23. Debbaut C, Vierendeels J, Casteleyn C, Cornillie P, Van Loo D, Simoens P, et al. Perfusion Characteristics of the Human Hepatic Microcirculation Based on Three-Dimensional Reconstructions and Computational Fluid Dynamic Analysis. J Biomech Eng (2012) 134:011003. doi: 10.1115/1.4005545
PubMed Abstract | CrossRef Full Text | Google Scholar
24. Nishigori N, Matsumoto M, Koyama F, Hayakawa M, Hatakeyayama K, Ko S, et al. Von Willebrand Factor-Rich Platelet Thrombi in the Liver Cause Sinusoidal Obstruction Syndrome Following Oxaliplatin-Based Chemotherapy. PloS One (2015) 10:e0143136. doi: 10.1371/journal.pone.0143136
PubMed Abstract | CrossRef Full Text | Google Scholar
25. Lemoinne S, Cadoret A, Rautou PE, El Mourabit H, Ratziu V, Corpechot C, et al. Portal Myofibroblasts Promote Vascular Remodeling Underlying Cirrhosis Formation Through the Release of Microparticles. Hepatology (2015) 61:1041–55. doi: 10.1002/hep.273182015
PubMed Abstract | CrossRef Full Text | Google Scholar
26. Zhu X, Han W, Wang L, Chu H, Zhao J, Xu Y, et al. Penicillar Arterioles of Red Pulp in Residual Spleen After Subtotal Splenectomy Due to Splenomegaly in Cirrhotic Patients: A Comparative Study. Int J Clin Exp Pathol (2015) 8:711–8.
PubMed Abstract | Google Scholar
27. Haier J, Korb T, Hotz B, Spiegel HU, Senninger N. An Intravital Model to Monitor Steps of Metastatic Tumor Cell Adhesion Within the Hepatic Microcirculation. J Gastrointest Surg (2003) 7:507–15. doi: 10.1016/S1091-255X(03)00023-4
PubMed Abstract | CrossRef Full Text | Google Scholar
28. von Sengbusch A, Gassmann P, Fisch KM, Enns A, Nicolson GL, Haier J. Focal Adhesion Kinase Regulates Metastatic Adhesion of Carcinoma Cells Within Liver Sinusoids. Am J Pathol (2005) 166:585–96. doi: 10.1016/S0002-9440(10)62280-8
PubMed Abstract | CrossRef Full Text | Google Scholar
29. Menter DG, Harkins C, Onoda J, Riorden W, Sloane BF, Taylor JD, et al. Inhibition of Tumor Cell Induced Platelet Aggregation by Prostacyclin and Carbacyclin: An Ultrastructural Study. Invasion Metastasis (1987) 7:109–28.
PubMed Abstract | Google Scholar
30. Crissman JD, Hatfield JS, Menter DG, Sloane B, Honn KV. Morphological Study of the Interaction of Intravascular Tumor Cells With Endothelial Cells and Subendothelial Matrix. Cancer Res (1988) 48:4065–72.
PubMed Abstract | Google Scholar
31. Walsh TG, Metharom P, Berndt MC. The Functional Role of Platelets in the Regulation of Angiogenesis. Platelets (2015) 26:199–211. doi: 10.3109/09537104.2014.909022
PubMed Abstract | CrossRef Full Text | Google Scholar
32. Kim KH, Barazia A, Cho J. Real-Time Imaging of Heterotypic Platelet-Neutrophil Interactions on the Activated Endothelium During Vascular Inflammation and Thrombus Formation in Live Mice. J Vis Exp: JoVE (2013). doi: 10.3791/50329
CrossRef Full Text | Google Scholar
33. Spectre G, Zhu L, Ersoy M, Hjemdahl P, Savion N, Varon D, et al. Platelets Selectively Enhance Lymphocyte Adhesion on Subendothelial Matrix Under Arterial Flow Conditions. Thromb Haemostasis (2012) 108:328–37. doi: 10.1160/TH12-02-0064
CrossRef Full Text | Google Scholar
34. Gardiner E, Andrews R. Platelet Adhesion. In: Gresele P, Kleiman N, Lopez J, Page C, editors. Platelets in Thrombotic and Non-Thrombotic Disorders. Switzerland: Springer International Publishing (2017). p. 309–19.
Google Scholar
35. Pothapragada S, Zhang P, Sheriff J, Livelli M, Slepian MJ, Deng Y, et al. A Phenomenological Particle-Based Platelet Model for Simulating Filopodia Formation During Early Activation. Int J Numer Method BioMed Eng (2015) 31:e02702. doi: 10.1002/cnm.2702
PubMed Abstract | CrossRef Full Text | Google Scholar
36. Kunert S, Meyer I, Fleischhauer S, Wannack M, Fiedler J, Shivdasani RA, et al. The Microtubule Modulator RanBP10 Plays a Critical Role in Regulation of Platelet Discoid Shape and Degranulation. Blood (2009) 114:5532–40. doi: 10.1182/blood-2009-04-216804
PubMed Abstract | CrossRef Full Text | Google Scholar
37. Jackson SP, Nesbitt WS, Westein E. Dynamics of Platelet Thrombus Formation. J Thromb Haemost (2009) 7 Suppl 1:17–20. doi: 10.1111/j.1538-7836.2009.03401.x
PubMed Abstract | CrossRef Full Text | Google Scholar
38. Italiano JE Jr., Bergmeier W, Tiwari S, Falet H, Hartwig JH, Hoffmeister KM, et al. Mechanisms and Implications of Platelet Discoid Shape. Blood (2003) 101:4789–96. doi: 10.1182/blood-2002-11-3491
PubMed Abstract | CrossRef Full Text | Google Scholar
39. Hartwig JH, Barkalow K, Azim A, Italiano J. The Elegant Platelet: Signals Controlling Actin Assembly. Thromb Haemostasis (1999) 82:392–8. doi: 10.1055/s-0037-1615858
CrossRef Full Text | Google Scholar
40. White JG, Rao GH. Microtubule Coils Versus the Surface Membrane Cytoskeleton in Maintenance and Restoration of Platelet Discoid Shape. Am J Pathol (1998) 152:597–609.
PubMed Abstract | Google Scholar
41. Polanowska-Grabowska R, Geanacopoulos M, Gear AR. Platelet Adhesion to Collagen via the Alpha 2 Beta 1 Integrin Under Arterial Flow Conditions Causes Rapid Tyrosine Phosphorylation of Pp125fak. Biochem J (1993) 296(Pt 3):543–7. doi: 10.1042/bj2960543
PubMed Abstract | CrossRef Full Text | Google Scholar
42. Falet H. Anatomy of the Platelet Cytoskeleton. In: Gresele P, Kleiman N, Lopez J, Page C, editors. Platelets in Thrombotic and Non-Thrombotic Disorders. Switzerland: Springer International Publishing (2017). p. 139–56.
Google Scholar
43. Lubkowska A, Dolegowska B, Banfi G. Growth Factor Content in PRP and Their Applicability in Medicine. J Biol Regul Homeost Agents (2012) 26:3S–22S.
PubMed Abstract | Google Scholar
44. Cakin MC, Ozdemir B, Kaya-Dagistanli F, Arkan H, Bahtiyar N, Anapali M, et al. Evaluation of the In Vivo Wound Healing Potential of the Lipid Fraction From Activated Platelet-Rich Plasma. Platelets (2020) 31:513–20. doi: 10.1080/09537104.2019.1663805
PubMed Abstract | CrossRef Full Text | Google Scholar
45. Etulain J. Platelets in Wound Healing and Regenerative Medicine. Platelets (2018) 29:556–68. doi: 10.1080/09537104.2018.1430357
PubMed Abstract | CrossRef Full Text | Google Scholar
46. Losi P, Barsotti MC, Foffa I, Buscemi M, De Almeida CV, Fabbri M, et al. In Vitro Human Cord Blood Platelet Lysate Characterisation With Potential Application in Wound Healing. Int Wound J (2019) 17:65–72. doi: 10.1111/iwj.13233
PubMed Abstract | CrossRef Full Text | Google Scholar
47. Myung H, Jang H, Myung JK, Lee C, Lee J, Kang J, et al. Platelet-Rich Plasma Improves the Therapeutic Efficacy of Mesenchymal Stem Cells by Enhancing Their Secretion of Angiogenic Factors in a Combined Radiation and Wound Injury Model. Exp Dermatol (2019) 29:158–67. doi: 10.1111/exd.14042
PubMed Abstract | CrossRef Full Text | Google Scholar
48. Battinelli EM, Thon JN, Okazaki R, Peters CG, Vijey P, Wilkie AR, et al. Megakaryocytes Package Contents Into Separate Alpha-Granules That are Differentially Distributed in Platelets. Blood Adv (2019) 3:3092–8. doi: 10.1182/bloodadvances.2018020834
PubMed Abstract | CrossRef Full Text | Google Scholar
49. Ambrosio AL, Di Pietro SM. Mechanism of Platelet Alpha-Granule Biogenesis: Study of Cargo Transport and the VPS33B-VPS16B Complex in a Model System. Blood Adv (2019) 3:2617–26. doi: 10.1182/bloodadvances.2018028969
PubMed Abstract | CrossRef Full Text | Google Scholar
50. Sharda A, Flaumenhaft R. The Life Cycle of Platelet Granules. F1000Res (2018) 7:236. doi: 10.12688/f1000research.13283.1
PubMed Abstract | CrossRef Full Text | Google Scholar
51. Thon JN, Peters CG, Machlus KR, Aslam R, Rowley J, Macleod H, et al. T Granules in Human Platelets Function in TLR9 Organization and Signaling. J Cell Biol (2012) 198:561–74. doi: 10.1083/jcb.201111136
PubMed Abstract | CrossRef Full Text | Google Scholar
52. Thon JN, Italiano JE. Platelet Formation. Semin Hematol (2010) 47:220–6. doi: 10.1053/j.seminhematol.2010.03.005
PubMed Abstract | CrossRef Full Text | Google Scholar
53. Flaumenhaft R, Dilks JR, Rozenvayn N, Monahan-Earley RA, Feng D, Dvorak AM. The Actin Cytoskeleton Differentially Regulates Platelet Alpha-Granule and Dense-Granule Secretion. Blood (2005) 105:3879–87. doi: 10.1182/blood-2004-04-1392
PubMed Abstract | CrossRef Full Text | Google Scholar
54. Koseoglu S, Dilks JR, Peters CG, Fitch-Tewfik JL, Fadel NA, Jasuja R, et al. Dynamin-Related Protein-1 Controls Fusion Pore Dynamics During Platelet Granule Exocytosis. Arterioscler Thromb Vasc Biol (2013) 33:481–8. doi: 10.1161/ATVBAHA.112.255737
PubMed Abstract | CrossRef Full Text | Google Scholar
55. Yadav S, Storrie B. The Cellular Basis of Platelet Secretion: Emerging Structure/Function Relationships. Platelets (2017) 28:108–18. doi: 10.1080/09537104.2016.1257786
PubMed Abstract | CrossRef Full Text | Google Scholar
56. Chen W, Tang D, Xu Y, Zou Y, Sui W, Dai Y, et al. Comprehensive Analysis of Lysine Crotonylation in Proteome of Maintenance Hemodialysis Patients. Med (Baltimore) (2018) 97:e12035. doi: 10.1097/MD.0000000000012035
CrossRef Full Text | Google Scholar
57. Stritt S, Beck S, Becker IC, Vogtle T, Hakala M, Heinze KG, et al. Twinfilin 2a Regulates Platelet Reactivity and Turnover in Mice. Blood (2017) 130:1746–56. doi: 10.1182/blood-2017-02-770768
PubMed Abstract | CrossRef Full Text | Google Scholar
58. Hui H, Fuller KA, Erber WN, Linden MD. Imaging Flow Cytometry in the Assessment of Leukocyte-Platelet Aggregates. Methods (2017) 112:46–54. doi: 10.1016/j.ymeth.2016.10.002
PubMed Abstract | CrossRef Full Text | Google Scholar
59. Lowe KL, Finney BA, Deppermann C, Hagerling R, Gazit SL, Frampton J, et al. Podoplanin and CLEC-2 Drive Cerebrovascular Patterning and Integrity During Development. Blood (2015) 125:3769–77. doi: 10.1182/blood-2014-09-603803
PubMed Abstract | CrossRef Full Text | Google Scholar
60. Qiu Y, Brown AC, Myers DR, Sakurai Y, Mannino RG, Tran R, et al. Platelet Mechanosensing of Substrate Stiffness During Clot Formation Mediates Adhesion, Spreading, and Activation. Proc Natl Acad Sci U.S.A. (2014) 111:14430–5. doi: 10.1073/pnas.1322917111
PubMed Abstract | CrossRef Full Text | Google Scholar
61. Menter DG, Tucker SC, Kopetz S, Sood AK, Crissman JD, Honn KV. Platelets and Cancer: A Casual or Causal Relationship: Revisited. Cancer Metastasis Rev (2014) 33:231–69. doi: 10.1007/s10555-014-9498-0
PubMed Abstract | CrossRef Full Text | Google Scholar
62. Peerschke EI, Reid KB, Ghebrehiwet B. Platelet Activation by C1q Results in the Induction of Alpha IIb/beta 3 Integrins (GPIIb-IIIa) and the Expression of P-Selectin and Procoagulant Activity. J Exp Med (1993) 178:579–87. doi: 10.1084/jem.178.2.579
PubMed Abstract | CrossRef Full Text | Google Scholar
63. Bevilacqua MP, Stengelin S, Gimbrone MA Jr., Seed B. Endothelial Leukocyte Adhesion Molecule 1: An Inducible Receptor for Neutrophils Related to Complement Regulatory Proteins and Lectins. Science (1989) 243:1160–5. doi: 10.1126/science.2466335
PubMed Abstract | CrossRef Full Text | Google Scholar
64. Menter DG, Steinert BW, Sloane BF, Gundlach N, O’Gara CY, Marnett LJ, et al. Role of Platelet Membrane in Enhancement of Tumor Cell Adhesion to Endothelial Cell Extracellular Matrix. Cancer Res (1987) 47:6751–62.
PubMed Abstract | Google Scholar
65. Menter DG, Sloane BF, Steinert BW, Onoda J, Craig R, Harkins C, et al. Platelet Enhancement of Tumor Cell Adhesion to Subendothelial Matrix: Role of Platelet Cytoskeleton and Platelet Membrane. J Natl Cancer Inst (1987) 79:1077–90.
PubMed Abstract | Google Scholar
66. Honn KV, Onoda JM, Menter DG, Cavanaugh PG, Taylor JD, Crissman JD, et al. Possible Strategies for Antimetastastic Therapy. Prog Clin Biol Res (1986) 212:217–49.
PubMed Abstract | Google Scholar
67. Martin-Granado V, Ortiz-Rivero S, Carmona R, Gutierrez-Herrero S, Barrera M, San-Segundo L, et al. C3G Promotes a Selective Release of Angiogenic Factors From Activated Mouse Platelets to Regulate Angiogenesis and Tumor Metastasis. Oncotarget (2017) 8:110994–1011. doi: 10.18632/oncotarget.22339
PubMed Abstract | CrossRef Full Text | Google Scholar
68. Shen EC, Chou TC, Gau CH, Tu HP, Chen YT, Fu E. Releasing Growth Factors From Activated Human Platelets After Chitosan Stimulation: A Possible Bio-Material for Platelet-Rich Plasma Preparation. Clin Oral Implants Res (2006) 17:572–8. doi: 10.1111/j.1600-0501.2004.01241.x
PubMed Abstract | CrossRef Full Text | Google Scholar
69. Murata S, Maruyama T, Nowatari T, Takahashi K, Ohkohchi N. Signal Transduction of Platelet-Induced Liver Regeneration and Decrease of Liver Fibrosis. Int J Mol Sci (2014) 15:5412–25. doi: 10.3390/ijms15045412
PubMed Abstract | CrossRef Full Text | Google Scholar
70. Starlinger P, Haegele S, Offensperger F, Oehlberger L, Pereyra D, Kral JB, et al. The Profile of Platelet Alpha-Granule Released Molecules Affects Postoperative Liver Regeneration. Hepatology (2016) 63:1675–88. doi: 10.1002/hep.28331
PubMed Abstract | CrossRef Full Text | Google Scholar
71. Spencer EM, Tokunaga A, Hunt TK. Insulin-Like Growth Factor Binding Protein-3 is Present in the Alpha-Granules of Platelets. Endocrinology (1993) 132:996–1001. doi: 10.1210/endo.132.3.7679986
PubMed Abstract | CrossRef Full Text | Google Scholar
72. Chan K, Spencer EM. Megakaryocytes Endocytose Insulin-Like Growth Factor (IGF) I and IGF-Binding Protein-3: A Novel Mechanism Directing Them Into Alpha Granules of Platelets. Endocrinology (1998) 139:559–65. doi: 10.1210/endo.139.2.5727
PubMed Abstract | CrossRef Full Text | Google Scholar
73. Capitano M, Zhao L, Cooper S, Thorsheim C, Suzuki A, Huang X, et al. Phosphatidylinositol Transfer Proteins Regulate Megakaryocyte TGF-Beta1 Secretion and Hematopoiesis in Mice. Blood (2018) 132:1027–38. doi: 10.1182/blood-2017-09-806257
PubMed Abstract | CrossRef Full Text | Google Scholar
74. Windelov NA, Ostrowski SR, Johansson PI, Wanscher M, Larsen CF, Sorensen AM, et al. Circulating Levels of Platelet Alpha-Granule Cytokines in Trauma Patients. Inflamm Res (2015) 64:235–41. doi: 10.1007/s00011-015-0802-4
PubMed Abstract | CrossRef Full Text | Google Scholar
75. Blakytny R, Ludlow A, Martin GE, Ireland G, Lund LR, Ferguson MW, et al. Latent TGF-Beta1 Activation by Platelets. J Cell Physiol (2004) 199:67–76. doi: 10.1002/jcp.10454
PubMed Abstract | CrossRef Full Text | Google Scholar
76. Gressner AM, Weiskirchen R, Breitkopf K, Dooley S. Roles of TGF-Beta in Hepatic Fibrosis. Front Biosci: J Virtual Libr (2002) 7:d793–807. doi: 10.2741/A812
CrossRef Full Text | Google Scholar
77. Puolakkainen P, Twardzik D, Ranchalis J, Moroni M, Mandeli J, Paciucci PA. Increase of Plasma Transforming Growth Factor Beta (TGF Beta) During Immunotherapy With IL-2. Cancer Invest (1995) 13:583–9. doi: 10.3109/07357909509024926
PubMed Abstract | CrossRef Full Text | Google Scholar
78. Fava RA, Casey TT, Wilcox J, Pelton RW, Moses HL, Nanney LB. Synthesis of Transforming Growth Factor-Beta 1 by Megakaryocytes and its Localization to Megakaryocyte and Platelet Alpha-Granules. Blood (1990) 76:1946–55. doi: 10.1182/blood.V76.10.1946.bloodjournal76101946
PubMed Abstract | CrossRef Full Text | Google Scholar
79. Assoian RK, Sporn MB. Type Beta Transforming Growth Factor in Human Platelets: Release During Platelet Degranulation and Action on Vascular Smooth Muscle Cells. J Cell Biol (1986) 102:1217–23. doi: 10.1083/jcb.102.4.1217
PubMed Abstract | CrossRef Full Text | Google Scholar
80. Akbulut H, Altuntas F, Akbulut KG, Ozturk G, Cindoruk M, Unal E, et al. Prognostic Role of Serum Vascular Endothelial Growth Factor, Basic Fibroblast Growth Factor and Nitric Oxide in Patients With Colorectal Carcinoma. Cytokine (2002) 20:184–90. doi: 10.1006/cyto.2002.1993
PubMed Abstract | CrossRef Full Text | Google Scholar
81. Frelinger AL 3rd, Gerrits AJ, Neculaes VB, Gremmel T, Torres AS, Caiafa A, et al. Tunable Activation of Therapeutic Platelet-Rich Plasma by Pulse Electric Field: Differential Effects on Clot Formation, Growth Factor Release, and Platelet Morphology. PloS One (2018) 13:e0203557. doi: 10.1371/journal.pone.0203557
PubMed Abstract | CrossRef Full Text | Google Scholar
82. Jonnalagadda D, Sunkara M, Morris AJ, Whiteheart SW. Granule-Mediated Release of Sphingosine-1-Phosphate by Activated Platelets. Biochim Biophys Acta (2014) 1841:1581–9. doi: 10.1016/j.bbalip.2014.08.013
PubMed Abstract | CrossRef Full Text | Google Scholar
83. Blair P, Flaumenhaft R. Platelet Alpha-Granules: Basic Biology and Clinical Correlates. Blood Rev (2009) 23:177–89. doi: 10.1016/j.blre.2009.04.001
PubMed Abstract | CrossRef Full Text | Google Scholar
84. Haemmerle M, Stone RL, Menter DG, Afshar-Kharghan V, Sood AK. The Platelet Lifeline to Cancer: Challenges and Opportunities. Cancer Cell (2018) 33:965–83. doi: 10.1016/j.ccell.2018.03.002
PubMed Abstract | CrossRef Full Text | Google Scholar
85. Menter DG, Kopetz S, Hawk E, Sood AK, Loree JM, Gresele P, et al. Platelet “First Responders” in Wound Response, Cancer, and Metastasis. Cancer Metastasis Rev (2017) 36:199–213. doi: 10.1007/s10555-017-9682-0
PubMed Abstract | CrossRef Full Text | Google Scholar
86. Borgognone A, Pulcinelli FM. Reduction of cAMP and cGMP Inhibitory Effects in Human Platelets by MRP4-Mediated Transport. Thromb Haemostasis (2012) 108:955–62. doi: 10.1160/TH12-04-0232
CrossRef Full Text | Google Scholar
87. Jedlitschky G, Tirschmann K, Lubenow LE, Nieuwenhuis HK, Akkerman JW, Greinacher A, et al. The Nucleotide Transporter MRP4 (ABCC4) is Highly Expressed in Human Platelets and Present in Dense Granules, Indicating a Role in Mediator Storage. Blood (2004) 104:3603–10. doi: 10.1182/blood-2003-12-4330
PubMed Abstract | CrossRef Full Text | Google Scholar
88. Israels SJ, McMillan-Ward EM. Palmitoylation Supports the Association of Tetraspanin CD63 With CD9 and Integrin Alphaiibbeta3 in Activated Platelets. Thromb Res (2010) 125:152–8. doi: 10.1016/j.thromres.2009.07.005
PubMed Abstract | CrossRef Full Text | Google Scholar
89. Israels SJ, McMillan-Ward EM, Easton J, Robertson C, McNicol A. CD63 Associates With the alphaIIb Beta3 Integrin-CD9 Complex on the Surface of Activated Platelets. Thromb Haemostasis (2001) 85:134–41. doi: 10.1055/s-0037-1612916
CrossRef Full Text | Google Scholar
90. Ciferri S, Emiliani C, Guglielmini G, Orlacchio A, Nenci GG, Gresele P. Platelets Release Their Lysosomal Content In Vivo in Humans Upon Activation. Thromb Haemostasis (2000) 83:157–64. doi: 10.1055/s-0037-1613772
CrossRef Full Text | Google Scholar
91. Sodergren AL, Ramstrom S. Detection of Lysosomal Exocytosis in Platelets by Flow Cytometry. Methods Mol Biol (2017) 1594:191–203. doi: 10.1007/978-1-4939-6934-0_12
PubMed Abstract | CrossRef Full Text | Google Scholar
92. Koseoglu S, Flaumenhaft R. Advances in Platelet Granule Biology. Curr Opin Hematol (2013) 20:464–71. doi: 10.1097/MOH.0b013e3283632e6b
PubMed Abstract | CrossRef Full Text | Google Scholar
93. Fukami MH, Salganicoff L. Human Platelet Storage Organelles. A Review. Thromb Haemostasis (1977) 38:963–70. doi: 10.1055/s-0038-1651914
CrossRef Full Text | Google Scholar
94. Tucci MA, Woodall J Jr, Asfour M, McGuire R, Benghuzzi HA. The Effects of Serotonin on Activated Macrophages - Biomed 2013. BioMed Sci Instrum (2013) 49:267–73.
PubMed Abstract | Google Scholar
95. King SM, McNamee RA, Houng AK, Patel R, Brands M, Reed GL. Platelet Dense-Granule Secretion Plays a Critical Role in Thrombosis and Subsequent Vascular Remodeling in Atherosclerotic Mice. Circulation (2009) 120:785–91. doi: 10.1161/CIRCULATIONAHA.108.845461
PubMed Abstract | CrossRef Full Text | Google Scholar
96. Menter DG, Onoda JM, Moilanen D, Sloane BF, Taylor JD, Honn KV. Inhibition by Prostacyclin of the Tumor Cell-Induced Platelet Release Reaction and Platelet Aggregation. J Natl Cancer Inst (1987) 78:961–9.
PubMed Abstract | Google Scholar
97. de las Casas-Engel M, Dominguez-Soto A, Sierra-Filardi E, Bragado R, Nieto C, Puig-Kroger A, et al. Serotonin Skews Human Macrophage Polarization Through HTR2B and HTR7. J Immunol (2013) 190:2301–10. doi: 10.4049/jimmunol.1201133
PubMed Abstract | CrossRef Full Text | Google Scholar
98. Chen Y, Yuan Y, Li W. Sorting Machineries: How Platelet-Dense Granules Differ From Alpha-Granules. Biosci Rep (2018) 38:1–9. doi: 10.1042/BSR20180458
CrossRef Full Text | Google Scholar
99. Graham GJ, Ren Q, Dilks JR, Blair P, Whiteheart SW, Flaumenhaft R. Endobrevin/VAMP-8-Dependent Dense Granule Release Mediates Thrombus Formation In Vivo. Blood (2009) 114:1083–90. doi: 10.1182/blood-2009-03-210211
PubMed Abstract | CrossRef Full Text | Google Scholar
100. Leitin VL, Missel’vits F, Liubimova EV, Domogatskii SP. The Role of Thrombocyte Prostanoids and Products Secreted by Dense Granules in the Platelet Attachment, Spreading and Aggregation on Collagen Substrates. Biokhimiia (1989) 54:1804–14.
PubMed Abstract | Google Scholar
101. Gleissner CA, von Hundelshausen P, Ley K. Platelet Chemokines in Vascular Disease. Arterioscler Thromb Vasc Biol (2008) 28:1920–7. doi: 10.1161/ATVBAHA.108.169417
PubMed Abstract | CrossRef Full Text | Google Scholar
102. Chatterjee M, Rath D, Gawaz M. Role of Chemokine Receptors CXCR4 and CXCR7 for Platelet Function. Biochem Soc Trans (2015) 43:720–6. doi: 10.1042/BST20150113
PubMed Abstract | CrossRef Full Text | Google Scholar
103. Chatterjee M, von Ungern-Sternberg SN, Seizer P, Schlegel F, Buttcher M, Sindhu NA, et al. Platelet-Derived CXCL12 Regulates Monocyte Function, Survival, Differentiation Into Macrophages and Foam Cells Through Differential Involvement of CXCR4-Cxcr7. Cell Death Dis (2015) 6:e1989. doi: 10.1038/cddis.2015.233
PubMed Abstract | CrossRef Full Text | Google Scholar
104. Brandt E, Petersen F, Ludwig A, Ehlert JE, Bock L, Flad HD. The Beta-Thromboglobulins and Platelet Factor 4: Blood Platelet-Derived CXC Chemokines With Divergent Roles in Early Neutrophil Regulation. J Leukoc Biol (2000) 67:471–8. doi: 10.1002/jlb.67.4.471
PubMed Abstract | CrossRef Full Text | Google Scholar
105. Mellembakken JR, Solum NO, Ueland T, Videm V, Aukrust P. Increased Concentrations of Soluble CD40 Ligand, RANTES and GRO-Alpha in Preeclampsia–Possible Role of Platelet Activation. Thromb Haemostasis (2001) 86:1272–6. doi: 10.1055/s-0037-1616061
CrossRef Full Text | Google Scholar
106. Mehrpouri M, Bashash D, Mohammadi MH, Gheydari ME, Satlsar ES, Hamidpour M. Co-Culture of Platelets With Monocytes Induced M2 Macrophage Polarization and Formation of Foam Cells: Shedding Light on the Crucial Role of Platelets in Monocyte Differentiation. Turk J Haematol (2019) 36:97–105. doi: 10.4274/tjh.galenos.2019.0218.0449
PubMed Abstract | CrossRef Full Text | Google Scholar
107. Heffron SP, Weinstock A, Scolaro B, Chen S, Sansbury BE, Marecki G, et al. Platelet-Conditioned Media Induces an Anti-Inflammatory Macrophage Phenotype Through EP4. J Thromb Haemost (2020) 19:562–73. doi: 10.1111/jth.15172
PubMed Abstract | CrossRef Full Text | Google Scholar
108. Guo L, Akahori H, Harari E, Smith SL, Polavarapu R, Karmali V, et al. CD163+ Macrophages Promote Angiogenesis and Vascular Permeability Accompanied by Inflammation in Atherosclerosis. J Clin Invest (2018) 128:1106–24.
PubMed Abstract | Google Scholar
109. Pinto ML, Rios E, Duraes C, Ribeiro R, Machado JC, Mantovani A, et al. The Two Faces of Tumor-Associated Macrophages and Their Clinical Significance in Colorectal Cancer. Front Immunol (2019) 10:1875. doi: 10.3389/fimmu.2019.01875
PubMed Abstract | CrossRef Full Text | Google Scholar
110. Anderson G, Rodriguez M, Reiter RJ. Multiple Sclerosis: Melatonin, Orexin, and Ceramide Interact With Platelet Activation Coagulation Factors and Gut-Microbiome-Derived Butyrate in the Circadian Dysregulation of Mitochondria in Glia and Immune Cells. Int J Mol Sci (2019) 20:5500–28. doi: 10.3390/ijms20215500
CrossRef Full Text | Google Scholar
111. Ascher S, Reinhardt C. The Gut Microbiota: An Emerging Risk Factor for Cardiovascular and Cerebrovascular Disease. Eur J Immunol (2018) 48:564–75. doi: 10.1002/eji.201646879
PubMed Abstract | CrossRef Full Text | Google Scholar
112. Sharma M, Mitnala S, Vishnubhotla RK, Mukherjee R, Reddy DN, Rao PN. The Riddle of Nonalcoholic Fatty Liver Disease: Progression From Nonalcoholic Fatty Liver to Nonalcoholic Steatohepatitis. J Clin Exp Hepatol (2015) 5:147–58. doi: 10.1016/j.jceh.2015.02.002
PubMed Abstract | CrossRef Full Text | Google Scholar
113. Harrison P, Cramer EM. Platelet Alpha-Granules. Blood Rev (1993) 7:52–62. doi: 10.1016/0268-960X(93)90024-X
PubMed Abstract | CrossRef Full Text | Google Scholar
114. Rijkers M, van den Eshof BL, van der Meer PF, van Alphen FPJ, de Korte D, Leebeek FWG, et al. Monitoring Storage Induced Changes in the Platelet Proteome Employing Label Free Quantitative Mass Spectrometry. Sci Rep (2017) 7:11045. doi: 10.1038/s41598-017-11643-w
PubMed Abstract | CrossRef Full Text | Google Scholar
115. Justo Sanz R, Monzon Manzano E, Fernandez Bello I, Teresa Alvarez Roman M, Martin Salces M, Rivas Pollmar MI, et al. Platelet Apoptosis and PAI-1 are Involved in the Pro-Coagulant State of Immune Thrombocytopaenia Patients Treated With Thrombopoietin Receptor Agonists. Thromb Haemostasis (2019) 119:645–59. doi: 10.1055/s-0039-1678706
CrossRef Full Text | Google Scholar
116. Chen TH, Hsu CM, Hsu HC, Chiu CT, Su MY, Chu YY, et al. Plasminogen Activator Inhibitor-1 is Associated With the Metabolism and Development of Advanced Colonic Polyps. Transl Res (2018) 200:43–53. doi: 10.1016/j.trsl.2018.05.010
PubMed Abstract | CrossRef Full Text | Google Scholar
117. Falvella FS, Cremolini C, Miceli R, Nichetti F, Cheli S, Antoniotti C, et al. Variant Alleles in Factor V, Prothrombin, Plasminogen Activator Inhibitor-1, Methylenetetrahydrofolate Reductase and Risk of Thromboembolism in Metastatic Colorectal Cancer Patients Treated With First-Line Chemotherapy Plus Bevacizumab. Pharmacogenomics J (2017) 17:331–6. doi: 10.1038/tpj.2016.22
PubMed Abstract | CrossRef Full Text | Google Scholar
118. Santos-Martinez MJ, Medina C, Jurasz P, Radomski MW. Role of Metalloproteinases in Platelet Function. Thromb Res (2008) 121:535–42. doi: 10.1016/j.thromres.2007.06.002
PubMed Abstract | CrossRef Full Text | Google Scholar
119. Mastenbroek TG, Feijge MA, Kremers RM, van den Bosch MT, Swieringa F, De Groef L, et al. Platelet-Associated Matrix Metalloproteinases Regulate Thrombus Formation and Exert Local Collagenolytic Activity. Arterioscler Thromb Vasc Biol (2015) 35:2554–61. doi: 10.1161/ATVBAHA.115.306153
PubMed Abstract | CrossRef Full Text | Google Scholar
120. Baker EA, Leaper DJ. Proteinases, Their Inhibitors, and Cytokine Profiles in Acute Wound Fluid. Wound Repair Regener (2000) 8:392–8. doi: 10.1111/j.1524-475X.2000.00392.x
CrossRef Full Text | Google Scholar
121. Bazan HE, Tao Y, Bazan NG. Platelet-Activating Factor Induces Collagenase Expression in Corneal Epithelial Cells. Proc Natl Acad Sci USA (1993) 90:8678–82. doi: 10.1073/pnas.90.18.8678
PubMed Abstract | CrossRef Full Text | Google Scholar
122. Tao Y, Bazan HE, Bazan NG. Platelet-Activating Factor Induces the Expression of Metalloproteinases-1 and -9, But Not -2 or -3, in the Corneal Epithelium. Invest Ophthalmol Vis Sci (1995) 36:345–54.
PubMed Abstract | Google Scholar
123. Borkham-Kamphorst E, Alexi P, Tihaa L, Haas U, Weiskirchen R. Platelet-Derived Growth Factor-D Modulates Extracellular Matrix Homeostasis and Remodeling Through TIMP-1 Induction and Attenuation of MMP-2 and MMP-9 Gelatinase Activities. Biochem Biophys Res Commun (2015) 457:307–13. doi: 10.1016/j.bbrc.2014.12.106
PubMed Abstract | CrossRef Full Text | Google Scholar
124. Peltonen R, Hagstrom J, Tervahartiala T, Sorsa T, Haglund C, Isoniemi H. High Expression of MMP-9 in Primary Tumors and High Preoperative MPO in Serum Predict Improved Prognosis in Colorectal Cancer With Operable Liver Metastases. Oncology (2020) 99:144–60. doi: 10.1159/000510609
PubMed Abstract | CrossRef Full Text | Google Scholar
125. Lee MA, Park JH, Rhyu SY, Oh ST, Kang WK, Kim HN. Wnt3a Expression is Associated With MMP-9 Expression in Primary Tumor and Metastatic Site in Recurrent or Stage IV Colorectal Cancer. BMC Cancer (2014) 14:125. doi: 10.1186/1471-2407-14-125
PubMed Abstract | CrossRef Full Text | Google Scholar
126. Ogawa M, Ikeuchi K, Watanabe M, Etoh K, Kobayashi T, Takao Y, et al. Expression of Matrix Metalloproteinase 7, Laminin and Type IV Collagen-Associated Liver Metastasis in Human Colorectal Cancer: Immunohistochemical Approach. Hepatogastroenterology (2005) 52:875–80.
PubMed Abstract | Google Scholar
127. Waas ET, Wobbes T, Lomme RM, DeGroot J, Ruers T, Hendriks T. Matrix Metalloproteinase 2 and 9 Activity in Patients With Colorectal Cancer Liver Metastasis. Br J Surg (2003) 90:1556–64. doi: 10.1002/bjs.4346
PubMed Abstract | CrossRef Full Text | Google Scholar
128. Paschos KA, Majeed AW, Bird NC. Natural History of Hepatic Metastases From Colorectal Cancer–Pathobiological Pathways With Clinical Significance. World J Gastroenterol (2014) 20:3719–37. doi: 10.3748/wjg.v20.i14.3719
PubMed Abstract | CrossRef Full Text | Google Scholar
129. Gordon JL. Blood Platelet Lysosomes and Their Contribution to the Pathophysiological Role of Platelets. Front Biol (1975) 43:3–31.
PubMed Abstract | Google Scholar
130. Misztal T, Rusak T, Branska-Januszewska J, Gasowska M, Szynaka B, Golaszewska A, et al. Aquaporins in Human Platelets: Intracellular Localization and Possible Role in Granule and Lysosome Secretion. Acta Biochim Pol (2018) 65:555–66. doi: 10.18388/abp.2018_2621
PubMed Abstract | CrossRef Full Text | Google Scholar
131. Nurden AT, Nurden P, Sanchez M, Andia I, Anitua E. Platelets and Wound Healing. Front Biosci: J Virtual Libr (2008) 13:3532–48. doi: 10.2741/2947
CrossRef Full Text | Google Scholar
132. Emiliani C, Martino S, Orlacchio A, Vezza R, Nenci GG, Gresele P. Platelet Glycohydrolase Activities: Characterization and Release. Cell Biochem Funct (1995) 13:31–9. doi: 10.1002/cbf.290130108
PubMed Abstract | CrossRef Full Text | Google Scholar
133. Metzelaar MJ, Clevers HC. Lysosomal Membrane Glycoproteins in Platelets. Thromb Haemostasis (1992) 68:378–82. doi: 10.1055/s-0038-1646280
CrossRef Full Text | Google Scholar
134. Waite M, Griffin HD. The Phospholipases A of Lysosomes. Front Biol (1976) 45:257–305.
PubMed Abstract | Google Scholar
135. Quach ME, Chen W, Li R. Mechanisms of Platelet Clearance and Translation to Improve Platelet Storage. Blood (2018) 131:1512–21. doi: 10.1182/blood-2017-08-743229
PubMed Abstract | CrossRef Full Text | Google Scholar
136. Hoffmeister KM, Falet H. Platelet Clearance by the Hepatic Ashwell-Morrell Receptor: Mechanisms and Biological Significance. Thromb Res (2016) 141 Suppl 2:S68–72. doi: 10.1016/S0049-3848(16)30370-X
PubMed Abstract | CrossRef Full Text | Google Scholar
137. Grozovsky R, Hoffmeister KM, Falet H. Novel Clearance Mechanisms of Platelets. Curr Opin Hematol (2010) 17:585–9. doi: 10.1097/MOH.0b013e32833e7561
PubMed Abstract | CrossRef Full Text | Google Scholar
138. Wang Y, Chen W, Zhang W, Lee-Sundlov MM, Casari C, Berndt MC, et al. Desialylation of O-Glycans on Glycoprotein Ibalpha Drives Receptor Signaling and Platelet Clearance. Haematologica (2020) 106:220–9. doi: 10.3324/haematol.2019.240440
CrossRef Full Text | Google Scholar
139. Dupont A, Soukaseum C, Cheptou M, Adam F, Nipoti T, Lourenco-Rodrigues MD, et al. Relevance of Platelet Desialylation and Thrombocytopenia in Type 2B Von Willebrand Disease: Preclinical and Clinical Evidence. Haematologica (2019) 104:2493–500. doi: 10.3324/haematol.2018.206250
PubMed Abstract | CrossRef Full Text | Google Scholar
140. Pereira-Neves A, Fragao-Marques M, Rocha-Neves J, Gamas L, Oliveira-Pinto J, Cerqueira A, et al. The Impact of Neutrophil-Tolymphocyte Ratio and Plateletto- Lymphocyte Ratio in Carotid Artery Disease. Rev Port Cir Cardiotorac Vasc (2021) 28:45–51.
PubMed Abstract | Google Scholar
141. Marques P, de Vries F, Dekkers OM, Korbonits M, Biermasz NR, Pereira AM. Serum Inflammation-Based Scores in Endocrine Tumors. J Clin Endocrinol Metab (2021). doi: 10.1210/clinem/dgab238
PubMed Abstract | CrossRef Full Text | Google Scholar
142. Kumar Gothwal S, Singh VB, Shrivastava M, Banseria R, Goyal K, Singh S, et al. Complete Blood-Count-Based Inflammatory Score (CBCS) of COVID-19 Patients at Tertiary Care Center. Altern Ther Health Med (2021) 27:18–24.
PubMed Abstract | Google Scholar
143. Ferro D, Matias M, Neto J, Dias R, Moreira G, Petersen N, et al. Neutrophil-To-Lymphocyte Ratio Predicts Cerebral Edema and Clinical Worsening Early After Reperfusion Therapy in Stroke. Stroke (2021) 52:859–67. doi: 10.1161/STROKEAHA.120.032130
PubMed Abstract | CrossRef Full Text | Google Scholar
144. Dong G, Huang A, Liu L. Platelet-To-Lymphocyte Ratio and Prognosis in STEMI: A Meta-Analysis. Eur J Clin Invest (2021) 51:e13386. doi: 10.1111/eci.13386
PubMed Abstract | CrossRef Full Text | Google Scholar
145. Alabraba E, Ibrahim H, Olaru A, Cameron I, Gomez D, Group NHS. Retrospective Cohort Study of Statin Therapy Effect on Resected Colorectal Liver Metastases. World J Gastrointest Surg (2020) 12:34–44. doi: 10.4240/wjgs.v12.i2.34
PubMed Abstract | CrossRef Full Text | Google Scholar
146. Gasparyan AY, Ayvazyan L, Mukanova U, Yessirkepov M, Kitas GD. The Platelet-To-Lymphocyte Ratio as an Inflammatory Marker in Rheumatic Diseases. Ann Lab Med (2019) 39:345–57. doi: 10.3343/alm.2019.39.4.345
PubMed Abstract | CrossRef Full Text | Google Scholar
147. Pravisani R, Mocchegiani F, Isola M, Lorenzin D, Adani GL, Cherchi V, et al. Postoperative Trends and Prognostic Values of Inflammatory and Nutritional Biomarkers After Liver Transplantation for Hepatocellular Carcinoma. Cancers (Basel) (2021) 13:513–27. doi: 10.3390/cancers13030513
PubMed Abstract | CrossRef Full Text | Google Scholar
148. Nylec M, Derbisz K, Chrzaszcz P, Wronska W, Krol R, Wystrychowski W. Preoperative Neutrophil-To-Lymphocyte Ratio as an Independent Predictor of 1-Year Graft Loss and Mortality After Orthotopic Liver Transplantation. Transplant Proc (2020) 52:2472–6. doi: 10.1016/j.transproceed.2020.03.036
PubMed Abstract | CrossRef Full Text | Google Scholar
149. Han S, Lee S, Yang JD, Leise MD, Ahn JH, Kim S, et al. Risk of Posttransplant Hepatocellular Carcinoma Recurrence is Greater in Recipients With Higher Platelet Counts in Living Donor Liver Transplantation. Liver Transpl (2018) 24:44–55. doi: 10.1002/lt.24961
PubMed Abstract | CrossRef Full Text | Google Scholar
150. Shen JY, Li C, Wen TF, Yan LN, Li B, Wang WT, et al. A Simple Prognostic Score System Predicts the Prognosis of Solitary Large Hepatocellular Carcinoma Following Hepatectomy. Med (Baltimore) (2016) 95:e4296. doi: 10.1097/MD.0000000000004296
CrossRef Full Text | Google Scholar
151. Lai Q, Castro Santa E, Rico Juri JM, Pinheiro RS, Lerut J. Neutrophil and Platelet-to-Lymphocyte Ratio as New Predictors of Dropout and Recurrence After Liver Transplantation for Hepatocellular Cancer. Transpl Int (2014) 27:32–41. doi: 10.1111/tri.12191
PubMed Abstract | CrossRef Full Text | Google Scholar
152. Peng HX, Lin K, He BS, Pan YQ, Ying HQ, Hu XX, et al. Platelet-To-Lymphocyte Ratio Could be a Promising Prognostic Biomarker for Survival of Colorectal Cancer: A Systematic Review and Meta-Analysis. FEBS Open Bio (2016) 6:742–50. doi: 10.1002/2211-5463.12083
PubMed Abstract | CrossRef Full Text | Google Scholar
153. Dingemans KP. A Morphological Study of the Invasion of Liver Tissue by Tumour Cells. Arch Chir Neerl (1973) 25:351–62.
PubMed Abstract | Google Scholar
154. Dingemans KP. Invasion of Liver Tissue by Blood-Borne Mammary Carcinoma Cells. J Natl Cancer Inst (1974) 53:1813–24. doi: 10.1093/jnci/53.6.1813
PubMed Abstract | CrossRef Full Text | Google Scholar
155. Zhou Z, Xu MJ, Gao B. Hepatocytes: A Key Cell Type for Innate Immunity. Cell Mol Immunol (2016) 13:301–15. doi: 10.1038/cmi.2015.97
PubMed Abstract | CrossRef Full Text | Google Scholar
156. Pant A, Kopec AK, Luyendyk JP. Role of the Blood Coagulation Cascade in Hepatic Fibrosis. Am J Physiol Gastrointest Liver Physiol (2018) 315:G171–6. doi: 10.1152/ajpgi.00402.2017
PubMed Abstract | CrossRef Full Text | Google Scholar
157. Luo DZ, Vermijlen D, Ahishali B, Triantis V, Plakoutsi G, Braet F, et al. On the Cell Biology of Pit Cells, the Liver-Specific NK Cells. World J Gastroenterol (2000) 6:1–11. doi: 10.3748/wjg.v6.i1.1
PubMed Abstract | CrossRef Full Text | Google Scholar
158. Luo DZ, Vermijlen D, Ahishali B, Triantis V, Vanderkerken K, Kuppen PJ, et al. Participation of CD45, NKR-P1A and ANK61 Antigen in Rat Hepatic NK Cell (Pit Cell)Mediated Target Cell Cytotoxicity. World J Gastroenterol (2000) 6:546–52.
PubMed Abstract | Google Scholar
159. Vermijlen D, Luo D, Froelich CJ, Medema JP, Kummer JA, Willems E, et al. Pit Cells Exclusively Kill P815 Tumor Cells by the Perforin/Granzyme Pathway. Comp Hepatol (2004) 3 Suppl 1:S58. doi: 10.1186/1476-5926-2-S1-S58
PubMed Abstract | CrossRef Full Text | Google Scholar
160. Wisse E, Luo D, Vermijlen D, Kanellopoulou C, De Zanger R, Braet F. On the Function of Pit Cells, the Liver-Specific Natural Killer Cells. Semin Liver Dis (1997) 17:265–86. doi: 10.1055/s-2007-1007204
PubMed Abstract | CrossRef Full Text | Google Scholar
161. Chwiki S, Campos MM, McLaughlin ME, Kleiner DE, Kovacs JA, Morse CG, et al. Adverse Effects of Antiretroviral Therapy on Liver Hepatocytes and Endothelium in HIV Patients: An Ultrastructural Perspective. Ultrastruct Pathol (2017) 41:186–95. doi: 10.1080/01913123.2017.1282066
PubMed Abstract | CrossRef Full Text | Google Scholar
162. Kong LJ, Li H, Du YJ, Pei FH, Hu Y, Zhao LL, et al. Vatalanib, a Tyrosine Kinase Inhibitor, Decreases Hepatic Fibrosis and Sinusoidal Capillarization in CCl4-Induced Fibrotic Mice. Mol Med Rep (2017) 15:2604–10. doi: 10.3892/mmr.2017.6325
PubMed Abstract | CrossRef Full Text | Google Scholar
163. McLean AJ, Cogger VC, Chong GC, Warren A, Markus AM, Dahlstrom JE, et al. Age-Related Pseudocapillarization of the Human Liver. J Pathol (2003) 200:112–7. doi: 10.1002/path.1328
PubMed Abstract | CrossRef Full Text | Google Scholar
164. Wack KE, Ross MA, Zegarra V, Sysko LR, Watkins SC, Stolz DB. Sinusoidal Ultrastructure Evaluated During the Revascularization of Regenerating Rat Liver. Hepatology (2001) 33:363–78. doi: 10.1053/jhep.2001.21998
PubMed Abstract | CrossRef Full Text | Google Scholar
165. Muerkoster S, Wachowski O, Zerban H, Schirrmacher V, Umansky V, Rocha M. Graft-Versus-Leukemia Reactivity Involves Cluster Formation Between Superantigen-Reactive Donor T Lymphocytes and Host Macrophages. Clin Cancer Res (1998) 4:3095–106.
PubMed Abstract | Google Scholar
166. Braet F, Vanbesien J, De Zanger R, Wisse E. Ageing of the Liver Sieve and Pseudocapillarisation. Lancet (2002) 360:1171–2. doi: 10.1016/S0140-6736(02)11214-1
PubMed Abstract | CrossRef Full Text | Google Scholar
167. Wisse E, Braet F, Luo D, De Zanger R, Jans D, Crabbe E, et al. Structure and Function of Sinusoidal Lining Cells in the Liver. Toxicol Pathol (1996) 24:100–11. doi: 10.1177/019262339602400114
PubMed Abstract | CrossRef Full Text | Google Scholar
168. Brenner DA, Kisseleva T, Scholten D, Paik YH, Iwaisako K, Inokuchi S, et al. Origin of Myofibroblasts in Liver Fibrosis. Fibrogenesis Tissue Repair (2012) 5:S17. doi: 10.1186/1755-1536-5-S1-S17
PubMed Abstract | CrossRef Full Text | Google Scholar
169. Strazzabosco M, Fabris L. Functional Anatomy of Normal Bile Ducts. Anat Rec (Hoboken) (2008) 291:653–60. doi: 10.1002/ar.20664
PubMed Abstract | CrossRef Full Text | Google Scholar
170. Zapotoczny B, Szafranska K, Kus E, Chlopicki S, Szymonski M. Quantification of Fenestrations in Liver Sinusoidal Endothelial Cells by Atomic Force Microscopy. Micron (2017) 101:48–53. doi: 10.1016/j.micron.2017.06.005
PubMed Abstract | CrossRef Full Text | Google Scholar
171. Wisse E, De Zanger RB, Jacobs R, McCuskey RS. Scanning Electron Microscope Observations on the Structure of Portal Veins, Sinusoids and Central Veins in Rat Liver. Scan Electron Microsc (1983) 3:1441–52.
Google Scholar
172. Poisson J, Lemoinne S, Boulanger C, Durand F, Moreau R, Valla D, et al. Liver Sinusoidal Endothelial Cells: Physiology and Role in Liver Diseases. J Hepatol (2017) 66:212–27. doi: 10.1016/j.jhep.2016.07.009
PubMed Abstract | CrossRef Full Text | Google Scholar
173. Chauhan A, Adams DH, Watson SP, Lalor PF. Platelets: No Longer Bystanders in Liver Disease. Hepatology (2016) 64:1774–84. doi: 10.1002/hep.28526
PubMed Abstract | CrossRef Full Text | Google Scholar
174. Hilscher MB, Sehrawat T, Arab JP, Zeng Z, Gao J, Liu M, et al. Mechanical Stretch Increases Expression of CXCL1 in Liver Sinusoidal Endothelial Cells to Recruit Neutrophils, Generate Sinusoidal Microthombi, and Promote Portal Hypertension. Gastroenterology (2019) 157:193–209 e9. doi: 10.1053/j.gastro.2019.03.013
PubMed Abstract | CrossRef Full Text | Google Scholar
175. Lalor PF, Herbert J, Bicknell R, Adams DH. Hepatic Sinusoidal Endothelium Avidly Binds Platelets in an Integrin-Dependent Manner, Leading to Platelet and Endothelial Activation and Leukocyte Recruitment. Am J Physiol Gastrointest Liver Physiol (2013) 304:G469–78. doi: 10.1152/ajpgi.00407.2012
PubMed Abstract | CrossRef Full Text | Google Scholar
176. Mielgo A, Schmid MC. Liver Tropism in Cancer: The Hepatic Metastatic Niche. Cold Spring Harb Perspect Med (2020) 10:1–18. doi: 10.1101/cshperspect.a037259
CrossRef Full Text | Google Scholar
177. Chang YZ, Yang L, Yang CQ. Migration of Hepatic Stellate Cells in Fibrotic Microenvironment of Diseased Liver Model. Hepatobiliary Pancreat Dis Int (2008) 7:401–5.
PubMed Abstract | Google Scholar
178. Haussinger D, Kordes C. Space of Disse: A Stem Cell Niche in the Liver. Biol Chem (2019) 401:81–95. doi: 10.1515/hsz-2019-0283
PubMed Abstract | CrossRef Full Text | Google Scholar
179. Ohtani O. Three-Dimensional Organization of the Collagen Fibrillar Framework of the Human and Rat Livers. Arch Histol Cytol (1988) 51:473–88. doi: 10.1679/aohc.51.473
PubMed Abstract | CrossRef Full Text | Google Scholar
180. Yang C, Zeisberg M, Mosterman B, Sudhakar A, Yerramalla U, Holthaus K, et al. Liver Fibrosis: Insights Into Migration of Hepatic Stellate Cells in Response to Extracellular Matrix and Growth Factors. Gastroenterology (2003) 124:147–59. doi: 10.1053/gast.2003.50012
PubMed Abstract | CrossRef Full Text | Google Scholar
181. Yang CQ, Yang L, Yang WZ, Zhang Z, Zhang H, Chang YZ, et al. Mechanism of Hepatic Stellate Cell Migration During Liver Fibrosis. Zhonghua Yi Xue Za Zhi (2008) 88:119–22.
PubMed Abstract | Google Scholar
182. Kakinuma S, Watanabe M. Analysis of the Mechanism Underlying Liver Diseases Using Human Induced Pluripotent Stem Cells. Immunol Med (2019) 42:71–8. doi: 10.1080/25785826.2019.1657254
PubMed Abstract | CrossRef Full Text | Google Scholar
183. Kholodenko IV, Kurbatov LK, Kholodenko RV, Manukyan GV, Yarygin KN. Mesenchymal Stem Cells in the Adult Human Liver: Hype or Hope? Cells (2019) 8:1127–64. doi: 10.3390/cells8101127
CrossRef Full Text | Google Scholar
184. Rong X, Yang Y, Zhang G, Zhang H, Li C, Wang Y. Antler Stem Cells as a Novel Stem Cell Source for Reducing Liver Fibrosis. Cell Tissue Res (2019) 379:195–206. doi: 10.1007/s00441-019-03081-z
PubMed Abstract | CrossRef Full Text | Google Scholar
185. Kordes C, Sawitza I, Gotze S, Herebian D, Haussinger D. Hepatic Stellate Cells Contribute to Progenitor Cells and Liver Regeneration. J Clin Invest (2014) 124:5503–15. doi: 10.1172/JCI74119
PubMed Abstract | CrossRef Full Text | Google Scholar
186. Wilkinson AL, Qurashi M, Shetty S. The Role of Sinusoidal Endothelial Cells in the Axis of Inflammation and Cancer Within the Liver. Front Physiol (2020) 11:990. doi: 10.3389/fphys.2020.00990
PubMed Abstract | CrossRef Full Text | Google Scholar
187. Kawada N, Tran-Thi TA, Klein H, Decker K. The Contraction of Hepatic Stellate (Ito) Cells Stimulated With Vasoactive Substances. Possible Involvement of Endothelin 1 and Nitric Oxide in the Regulation of the Sinusoidal Tonus. Eur J Biochem (1993) 213:815–23. doi: 10.1111/j.1432-1033.1993.tb17824.x
PubMed Abstract | CrossRef Full Text | Google Scholar
188. Roos E, Dingemans KP, Van de Pavert IV, Van den Bergh-Weerman MA. Mammary-Carcinoma Cells in Mouse Liver: Infiltration of Liver Tissue and Interaction With Kupffer Cells. Br J Cancer (1978) 38:88–99. doi: 10.1038/bjc.1978.167
PubMed Abstract | CrossRef Full Text | Google Scholar
189. Halpern KB, Shenhav R, Matcovitch-Natan O, Toth B, Lemze D, Golan M, et al. Single-Cell Spatial Reconstruction Reveals Global Division of Labour in the Mammalian Liver. Nature (2017) 542:352–6. doi: 10.1038/nature21065
PubMed Abstract | CrossRef Full Text | Google Scholar
190. Halpern KB, Shenhav R, Matcovitch-Natan O, Toth B, Lemze D, Golan M, et al. Erratum: Single-Cell Spatial Reconstruction Reveals Global Division of Labour in the Mammalian Liver. Nature (2017) 543:742. doi: 10.1038/nature21729
PubMed Abstract | CrossRef Full Text | Google Scholar
191. Gebhardt R. Metabolic Zonation of the Liver: Regulation and Implications for Liver Function. Pharmacol Ther (1992) 53:275–354. doi: 10.1016/0163-7258(92)90055-5
PubMed Abstract | CrossRef Full Text | Google Scholar
192. Kietzmann T. Metabolic Zonation of the Liver: The Oxygen Gradient Revisited. Redox Biol (2017) 11:622–30. doi: 10.1016/j.redox.2017.01.012
PubMed Abstract | CrossRef Full Text | Google Scholar
193. MacParland SA, Liu JC, Ma XZ, Innes BT, Bartczak AM, Gage BK, et al. Single Cell RNA Sequencing of Human Liver Reveals Distinct Intrahepatic Macrophage Populations. Nat Commun (2018) 9:4383. doi: 10.1038/s41467-018-06318-7
PubMed Abstract | CrossRef Full Text | Google Scholar
194. Amitrano L, Guardascione MA, Brancaccio V, Balzano A. Coagulation Disorders in Liver Disease. Semin Liver Dis (2002) 22:83–96. doi: 10.1055/s-2002-23205
PubMed Abstract | CrossRef Full Text | Google Scholar
195. Kopec AK, Luyendyk JP. Coagulation in Liver Toxicity and Disease: Role of Hepatocyte Tissue Factor. Thromb Res (2014) 133 Suppl 1:S57–9. doi: 10.1016/j.thromres.2014.03.023
PubMed Abstract | CrossRef Full Text | Google Scholar
196. Mucino-Bermejo J, Carrillo-Esper R, Uribe M, Mendez-Sanchez N. Coagulation Abnormalities in the Cirrhotic Patient. Ann Hepatol (2013) 12:713–24. doi: 10.1016/S1665-2681(19)31312-2
PubMed Abstract | CrossRef Full Text | Google Scholar
197. Arruda VR, Samelson-Jones BJ. Obstacles and Future of Gene Therapy for Hemophilia. Expert Opin Orphan Drugs (2015) 3:997–1010. doi: 10.1517/21678707.2015.1069179
PubMed Abstract | CrossRef Full Text | Google Scholar
198. Roberts HR, Cederbaum AI. The Liver and Blood Coagulation: Physiology and Pathology. Gastroenterology (1972) 63:297–320. doi: 10.1016/S0016-5085(19)33318-9
PubMed Abstract | CrossRef Full Text | Google Scholar
199. Kelly DA, Tuddenham EG. Haemostatic Problems in Liver Disease. Gut (1986) 27:339–49. doi: 10.1136/gut.27.3.339
PubMed Abstract | CrossRef Full Text | Google Scholar
200. Green G, Poller L, Thomson JM, Dymock IW. Factor VII as a Marker of Hepatocellular Synthetic Function in Liver Disease. J Clin Pathol (1976) 29:971–5. doi: 10.1136/jcp.29.11.971
PubMed Abstract | CrossRef Full Text | Google Scholar
201. La Mura V, Reverter JC, Flores-Arroyo A, Raffa S, Reverter E, Seijo S, et al. Von Willebrand Factor Levels Predict Clinical Outcome in Patients With Cirrhosis and Portal Hypertension. Gut (2011) 60:1133–8. doi: 10.1136/gut.2010.235689
PubMed Abstract | CrossRef Full Text | Google Scholar
202. Peck-Radosavljevic M. Review Article: Coagulation Disorders in Chronic Liver Disease. Aliment Pharmacol Ther (2007) 26 Suppl 1:21–8. doi: 10.1111/j.1365-2036.2007.03509.x
PubMed Abstract | CrossRef Full Text | Google Scholar
203. Huang H, Tohme S, Al-Khafaji AB, Tai S, Loughran P, Chen L, et al. Damage-Associated Molecular Pattern-Activated Neutrophil Extracellular Trap Exacerbates Sterile Inflammatory Liver Injury. Hepatology (2015) 62:600–14. doi: 10.1002/hep.27841
PubMed Abstract | CrossRef Full Text | Google Scholar
204. Dal-Secco D, Wang J, Zeng Z, Kolaczkowska E, Wong CH, Petri B, et al. A Dynamic Spectrum of Monocytes Arising From the in Situ Reprogramming of CCR2+ Monocytes at a Site of Sterile Injury. J Exp Med (2015) 212:447–56. doi: 10.1084/jem.20141539
PubMed Abstract | CrossRef Full Text | Google Scholar
205. Clark SR, Ma AC, Tavener SA, McDonald B, Goodarzi Z, Kelly MM, et al. Platelet TLR4 Activates Neutrophil Extracellular Traps to Ensnare Bacteria in Septic Blood. Nat Med (2007) 13:463–9. doi: 10.1038/nm1565
PubMed Abstract | CrossRef Full Text | Google Scholar
206. Sakurai K, Miyashita T, Okazaki M, Yamaguchi T, Ohbatake Y, Nakanuma S, et al. Role for Neutrophil Extracellular Traps (NETs) and Platelet Aggregation in Early Sepsis-Induced Hepatic Dysfunction. Vivo (2017) 31:1051–8. doi: 10.21873/invivo.11169
CrossRef Full Text | Google Scholar
207. Bullman S, Pedamallu CS, Sicinska E, Clancy TE, Zhang X, Cai D, et al. Analysis of Fusobacterium Persistence and Antibiotic Response in Colorectal Cancer. Science (2017) 358:1443–8.
PubMed Abstract | Google Scholar
208. Bjornson HS, Hill EO. Bacteroidaceae in Thromboembolic Disease: Effects of Cell Wall Components on Blood Coagulation In Vivo and In Vitro. Infect Immun (1973) 8:911–8. doi: 10.1128/iai.8.6.911-918.1973
PubMed Abstract | CrossRef Full Text | Google Scholar
209. Forrester LJ, Campbell BJ, Berg JN, Barrett JT. Aggregation of Platelets by Fusobacterium Necrophorum. J Clin Microbiol (1985) 22:245–9. doi: 10.1128/jcm.22.2.245-249.1985
PubMed Abstract | CrossRef Full Text | Google Scholar
210. Alkim H, Ayaz S, Sasmaz N, Oguz P, Sahin B. Hemostatic Abnormalities in Cirrhosis and Tumor-Related Portal Vein Thrombosis. Clin Appl Thromb Hemost (2012) 18:409–15. doi: 10.1177/1076029611427900
PubMed Abstract | CrossRef Full Text | Google Scholar
211. Ciancio G, Soloway M. Renal Cell Carcinoma Invading the Hepatic Veins. Cancer (2001) 92:1836–42. doi: 10.1002/1097-0142(20011001)92:7<1836::AID-CNCR1700>3.0.CO;2-R
PubMed Abstract | CrossRef Full Text | Google Scholar
212. Hurtado-Cordovi J, Lipka S, Avezbakiyev B, Multz AS. Budd-Chiari Syndrome Induced by Stage IV Rectal Carcinoid. Am J Med Sci (2013) 345:246–7. doi: 10.1097/MAJ.0b013e318271fb4f
PubMed Abstract | CrossRef Full Text | Google Scholar
213. Bikdeli B, Jimenez D, Garcia-Tsao G, Barba R, Font C, Diaz-Pedroche MDC, et al. Venous Thromboembolism in Patients With Liver Cirrhosis: Findings From the RIETE (Registro Informatizado De La Enfermedad TromboEmbolica) Registry. Semin Thromb Hemostasis (2019) 45:793–801. doi: 10.1055/s-0039-1697682
CrossRef Full Text | Google Scholar
214. Kujovich JL. Hemostatic Defects in End Stage Liver Disease. Crit Care Clinics (2005) 21:563–87. doi: 10.1016/j.ccc.2005.03.002
CrossRef Full Text | Google Scholar
215. Bahirwani R, Reddy KR. Drug-Induced Liver Injury Due to Cancer Chemotherapeutic Agents. Semin Liver Dis (2014) 34:162–71. doi: 10.1055/s-0034-1375957
PubMed Abstract | CrossRef Full Text | Google Scholar
216. Thatishetty AV, Agresti N, O’Brien CB. Chemotherapy-Induced Hepatotoxicity. Clinics Liver Dis (2013) 17:671–86, ix-x. doi: 10.1016/j.cld.2013.07.010
CrossRef Full Text | Google Scholar
217. Vincenzi B, Armento G, Spalato Ceruso M, Catania G, Leakos M, Santini D, et al. Drug-Induced Hepatotoxicity in Cancer Patients - Implication for Treatment. Expert Opin Drug Saf (2016) 15:1219–38. doi: 10.1080/14740338.2016.1194824
PubMed Abstract | CrossRef Full Text | Google Scholar
218. Carpino G, Del Ben M, Pastori D, Carnevale R, Baratta F, Overi D, et al. Increased Liver Localization of Lipopolysaccharides in Human and Experimental non-Alcoholic Fatty Liver Disease. Hepatology (2019) 72:470–85. doi: 10.1002/hep.31056
CrossRef Full Text | Google Scholar
219. Ogasawara F, Fusegawa H, Haruki Y, Shiraishi K, Watanabe N, Matsuzaki S. Platelet Activation in Patients With Alcoholic Liver Disease. Tokai J Exp Clin Med (2005) 30:41–8.
PubMed Abstract | Google Scholar
220. Shao JF, Zhan QG, Liu ZM, Zhong YG, Guan YL, Fu JP, et al. [Significance of Detecting Platelet Associated Antibody and Platelet Membrane Glycoprotein for Diagnosis of Immune Thrombocytopenia]. Zhongguo Shi Yan Xue Ye Xue Za Zhi (2004) 12:224–7.
PubMed Abstract | Google Scholar
221. Tajima H, Ohta T, Miyashita T, Nakanuma S, Matoba M, Miyata T, et al. Oxaliplatin-Based Chemotherapy Induces Extravasated Platelet Aggregation in the Liver. Mol Clin Oncol (2015) 3:555–8. doi: 10.3892/mco.2015.512
PubMed Abstract | CrossRef Full Text | Google Scholar
222. Nakano H, Oussoultzoglou E, Rosso E, Casnedi S, Chenard-Neu MP, Dufour P, et al. Sinusoidal Injury Increases Morbidity After Major Hepatectomy in Patients With Colorectal Liver Metastases Receiving Preoperative Chemotherapy. Ann Surg (2008) 247:118–24. doi: 10.1097/SLA.0b013e31815774de
PubMed Abstract | CrossRef Full Text | Google Scholar
223. Zen Y, Yeh MM. Checkpoint Inhibitor-Induced Liver Injury: A Novel Form of Liver Disease Emerging in the Era of Cancer Immunotherapy. Semin Diagn Pathol (2019) 36:434–40. doi: 10.1053/j.semdp.2019.07.009
PubMed Abstract | CrossRef Full Text | Google Scholar
224. Abdol Razak NB, Jones G, Bhandari M, Berndt MC, Metharom P. Cancer-Associated Thrombosis: An Overview of Mechanisms, Risk Factors, and Treatment. Cancers (Basel) (2018) 10:380–401. doi: 10.3390/cancers10100380
CrossRef Full Text | Google Scholar
225. Kanikarla-Marie P, Kopetz S, Hawk ET, Millward SW, Sood AK, Gresele P, et al. Bioactive Lipid Metabolism in Platelet “First Responder” and Cancer Biology. Cancer Metastasis Rev (2018) 37:439–54. doi: 10.1007/s10555-018-9755-8
PubMed Abstract | CrossRef Full Text | Google Scholar
226. Kanikarla-Marie P, Lam M, Sorokin AV, Overman MJ, Kopetz S, Menter DG. Platelet Metabolism and Other Targeted Drugs; Potential Impact on Immunotherapy. Front Oncol (2018) 8:107. doi: 10.3389/fonc.2018.00107
PubMed Abstract | CrossRef Full Text | Google Scholar
227. Khorana AA, Dalal M, Lin J, Connolly GC. Incidence and Predictors of Venous Thromboembolism (VTE) Among Ambulatory High-Risk Cancer Patients Undergoing Chemotherapy in the United States. Cancer (2013) 119:648–55. doi: 10.1002/cncr.27772
PubMed Abstract | CrossRef Full Text | Google Scholar
228. Behling K, Maguire WF, Di Gialleonardo V, Heeb LE, Hassan IF, Veach DR, et al. Remodeling the Vascular Microenvironment of Glioblastoma With Alpha-Particles. J Nucl Med (2016) 57:1771–7. doi: 10.2967/jnumed.116.173559
PubMed Abstract | CrossRef Full Text | Google Scholar
229. Sobolewska B, Grimmel C, Gatsiou A, Sopova K, Klein J, Biedermann T, et al. Different Effects of Ranibizumab and Bevacizumab on Platelet Activation Profile. Ophthalmologica. Journal International D’ophtalmologie. Int J Ophthalmol Z fur Augenheilkunde (2015) 234:195–210. doi: 10.1159/000437057
CrossRef Full Text | Google Scholar
230. Touyz RM, Herrmann SMS, Herrmann J. Vascular Toxicities With VEGF Inhibitor Therapies-Focus on Hypertension and Arterial Thrombotic Events. J Am Soc Hypertens (2018) 12:409–25. doi: 10.1016/j.jash.2018.03.008
PubMed Abstract | CrossRef Full Text | Google Scholar
231. Cui S, Shibamoto T, Liu W, Takano H, Kurata Y. Effects of Platelet-Activating Factor, Thromboxane A2 and Leukotriene D4 on Isolated Perfused Rat Liver. Prostaglandins Other Lipid Mediators (2006) 80:35–45. doi: 10.1016/j.prostaglandins.2006.03.004
PubMed Abstract | CrossRef Full Text | Google Scholar
232. Ruan Z, Shibamoto T, Shimo T, Koizumi T, Tsuchida H, Kurata Y, et al. Effects of Platelet-Activating Factor and Thromboxane A2 on Isolated Perfused Guinea Pig Liver. Prostaglandins Other Lipid Mediators (2004) 73:73–85. doi: 10.1016/j.prostaglandins.2003.11.002
PubMed Abstract | CrossRef Full Text | Google Scholar
233. Riedl J, Ay C. Venous Thromboembolism in Brain Tumors: Risk Factors, Molecular Mechanisms, and Clinical Challenges. Semin Thromb Hemostasis (2019) 45:334–41. doi: 10.1055/s-0039-1688493
CrossRef Full Text | Google Scholar
234. Tullemans BME, Heemskerk JWM, Kuijpers MJE. Acquired Platelet Antagonism: Off-Target Antiplatelet Effects of Malignancy Treatment With Tyrosine Kinase Inhibitors. J Thromb Haemost (2018) 16:1686–99. doi: 10.1111/jth.14225
PubMed Abstract | CrossRef Full Text | Google Scholar
235. Baaten C, Moenen F, Henskens YMC, Swieringa F, Wetzels RJH, van Oerle R, et al. Impaired Mitochondrial Activity Explains Platelet Dysfunction in Thrombocytopenic Cancer Patients Undergoing Chemotherapy. Haematologica (2018) 103:1557–67. doi: 10.3324/haematol.2017.185165
PubMed Abstract | CrossRef Full Text | Google Scholar
236. Riedl J, Kaider A, Marosi C, Prager GW, Eichelberger B, Assinger A, et al. Decreased Platelet Reactivity in Patients With Cancer is Associated With High Risk of Venous Thromboembolism and Poor Prognosis. Thromb Haemostasis (2017) 117:90–8. doi: 10.1160/TH16-02-0123
CrossRef Full Text | Google Scholar
237. Baaten CC, Moenen FC, Henskens YM, Swieringa F, Wetzels R, van Oerle R, et al. OC-08 - Multiple Functional Defects in Platelets From Thrombocytopenic Cancer Patients Undergoing Chemotherapy. Thromb Res (2016) 140 Suppl 1:S171. doi: 10.1016/S0049-3848(16)30125-6
PubMed Abstract | CrossRef Full Text | Google Scholar
238. Kissova J, Bulikova A, Ovesna P, Bourkova L, Penka M. Increased Mean Platelet Volume and Immature Platelet Fraction as Potential Predictors of Thrombotic Complications in BCR/ABL-Negative Myeloproliferative Neoplasms. Int J Hematol (2014) 100:429–36. doi: 10.1007/s12185-014-1673-0
PubMed Abstract | CrossRef Full Text | Google Scholar
239. Milosevic I, Vujovic A, Barac A, Djelic M, Korac M, Radovanovic Spurnic A, et al. Gut Microbiota, and Its Modulation in the Management of Liver Diseases: A Review of the Literature. Int J Mol Sci (2019) 20:395–411. doi: 10.3390/ijms20020395
CrossRef Full Text | Google Scholar
240. Komaki Y, Komaki F, Micic D, Ido A, Sakuraba A. Risk of Colorectal Cancer in Chronic Liver Diseases: A Systematic Review and Meta-Analysis. Gastrointest Endosc (2017) 86:93–104.e5. doi: 10.1016/j.gie.2016.12.009
PubMed Abstract | CrossRef Full Text | Google Scholar
241. Levitan N, Dowlati A, Remick SC, Tahsildar HI, Sivinski LD, Beyth R, et al. Rates of Initial and Recurrent Thromboembolic Disease Among Patients With Malignancy Versus Those Without Malignancy. Risk Analysis Using Medicare Claims Data. Med (Baltimore) (1999) 78:285–91. doi: 10.1097/00005792-199909000-00001
CrossRef Full Text | Google Scholar
242. Seretis C, Youssef H, Chapman M. Hypercoagulation in Colorectal Cancer: What can Platelet Indices Tell Us? Platelets (2015) 26:114–8. doi: 10.3109/09537104.2014.894969
PubMed Abstract | CrossRef Full Text | Google Scholar
243. Steele G Jr., Ravikumar TS. Resection of Hepatic Metastases From Colorectal Cancer. Biologic perspective. Ann Surg (1989) 210:127–38. doi: 10.1097/00000658-198908000-00001
PubMed Abstract | CrossRef Full Text | Google Scholar
244. Montomoli J, Erichsen R, Christiansen CF, Ulrichsen SP, Pedersen L, Nilsson T, et al. Liver Disease and 30-Day Mortality After Colorectal Cancer Surgery: A Danish Population-Based Cohort Study. BMC Gastroenterol (2013) 13:66. doi: 10.1186/1471-230X-13-66
PubMed Abstract | CrossRef Full Text | Google Scholar
245. Sorensen HT, Friis S, Olsen JH, Thulstrup AM, Mellemkjaer L, Linet M, et al. Risk of Breast Cancer in Men With Liver Cirrhosis. Am J Gastroenterol (1998) 93:231–3. doi: 10.1111/j.1572-0241.1998.00231.x
PubMed Abstract | CrossRef Full Text | Google Scholar
246. Kley HK, Deselaers T, Peerenboom H, Kruskemper HL. Enhanced Conversion of Androstenedione to Estrogens in Obese Males. J Clin Endocrinol Metab (1980) 51:1128–32. doi: 10.1210/jcem-51-5-1128
PubMed Abstract | CrossRef Full Text | Google Scholar
247. Longcope C, Pratt JH, Schneider S, Fineberg E. Estrogen and Androgen Dynamics in Liver Disease. J Endocrinol Invest (1984) 7:629–34. doi: 10.1007/BF03349497
PubMed Abstract | CrossRef Full Text | Google Scholar
248. Pignata S, Daniele B, Galati MG, Esposito G, Vallone P, Fiore F, et al. Oestradiol and Testosterone Blood Levels in Patients With Viral Cirrhosis and Hepatocellular Carcinoma. Eur J Gastroenterol Hepatol (1997) 9:283–6. doi: 10.1097/00042737-199703000-00012
PubMed Abstract | CrossRef Full Text | Google Scholar
Last Updated:
![]() ![]() ![]() |
Platelet α-Granules
Of the various types of platelet granules, the most common are α-granules, which constitute 10% of the platelet volume and number 50-80 per platelet (52). Two pathways contribute to α-granule formation. De novo synthesis occurs when the trans-Golgi network generates a core structure that attracts clathrin to form a lattice structure and interacts with coat proteins like adaptor-protein 1 resulting in clathrin-coated pits. After invagination, these pits bud forming membrane-bound vesicles that end up in early endosomes. A strictly endocytic vesicle pathway also exists involving adaptor-protein 2 that is involved in forming subsets of endosomes. Alpha-granule subset lineages mature in multivesicular bodies through the engagement of vacuolar protein sorting-associated protein (-33B and -16B) that are involved in sorting certain cargo from the trans-Golgi network. Neurobeachin-like protein 2 is another molecule that helps drive α-granule development and secretion. Mature α-granules are released via exocytosis following platelet stimulation via a process involving dynamin-related protein-1 and other cytoskeletal elements (53–55).
Author Contributions
PK and NF contributed equally. All authors contributed to the article and approved the submitted version.
Nueva Codere to match pre-pandemic 2019 results
Nueva Codere has outlined that its restructured business is on course to surpass its 2019 …
Betway hit with £400k penalty for West Ham marketing
September 23, 2022
Hemostasis, Chronic Trauma, and Cancer
Trauma triggers clotting mechanisms that prevent excessive blood loss from organs and the body in general. The initial hemodynamic response to trauma is contractions in vessel walls of smooth muscle resulting in vasoconstriction to limit blood loss—a response that is highly transient. Fortunately, vasoconstriction of damaged blood vessels is concomitant with the initiation of the coagulation process. Indeed, trauma also induces exposure of platelets to prothrombogenic extracellular matrix and tissue factor (i.e. platelet tissue factor, tissue thromboplastin, factor III, or CD142). The associated mechanisms of clot formation in primary hemostasis include platelet activation, adhesion, and aggregation resulting in platelet micro-plug formation. Secondary homeostasis includes strengthening of the expanding plug—a process that involves clotting factors—and formation of fibrin (1). Wound healing, like cancer, involves the invasion of immune cells, fibroblasts, and other stromal cells to cleanse and repair the lesion (2–4). During the acute phase of wound healing, the subsequent repair and resolution involves the additional action of anticoagulant proteins to break down the fibrin mesh. Once sufficient healing of the wound has occurred, a clot dissolution process takes place with the activation of plasminogen. In the case of chronic inflammation associated with non-alcoholic steatohepatitis (NASH), other liver diseases (5), or cancer, the resolution phase stalls or is suppressed by the non-healing abnormal tissue (2–4). Each organ has unique endogenous cellular characteristics and tissue matrices that govern interactions of coagulation and immune responses. The liver contains a unique microenvironment with a heterogeneous mix of many cell types (6). This unique cellular complexity provides distinctive biological influences on tissue wounding and repair (7). While the intricacies of this unique liver biology and its interface with platelets is not well understood, platelets do help in the infiltration of immune cells by initiating the tumor immune response (8).
Getting to the top of the funnel – DAZN, LiveScore and WSC debate betting and media
September 22, 2022
Building the UK’s data infrastructure
This supports work that is already underway, across government, to improve how data is used. Data has become a part of our core national infrastructure, and a huge driver of innovation. Countries where businesses and public services have reliable access to trusted data reap similar benefits to countries that led the world with access to transport, water and sewage infrastructure in previous centuries.
Registers, canonical lists of core reference data, are at the forefront of the government’s effort. They enable a standardised way of storing and accessing data, independently of the technology platforms and digital services that use them. Government’s expectation is that over time they will form the basis of government’s data infrastructure, helping people put government data to work.
Funding
DM is supported by Institutional Research Grant from UT MD Anderson Cancer Center. SK, DM, PKM, AD, JS, NY, and MO are supported by the Colorectal Cancer Moon Shot. SK, DM, JS and MO are supported by 2P30CA016672-43, 1P50CA221707-01A1, 5R01CA218230-02 and 5R01CA184843-05. AKS is supported by P50 CA217685, R35 CA209904, the American Cancer Society, and the Frank McGraw Memorial Chair in Cancer Research. PK is supported by Cancer Prevention Research Training Program, the Janice Davis Gordon Memorial Postdoctoral Fellowship in Colorectal Cancer Prevention Research, University of Texas MD Anderson Cancer Center. VAK is supported by the National Cancer Institute (CA231141, CA177909) and McCullough Professorship for Cancer Research. JS is supported by the Cancer Prevention & Research Institute of Texas as a CPRIT Scholar in Cancer Research (RR180035) and the National Cancer Institute (L30 CA171000 and K22 CA234406).
α-Granule Adhesion and Coagulation Molecules
Adhesion proteins released from α-granules help to stimulate the rapid arrest of platelets in circulation mediated by vonWillebrand factor (vWF), fibrinogen, integrins (αIIbβ3 and αvβ3), P–selectin, thrombospondin, and fibronectin. These proteins regulate interactions between platelets and endothelial cells, exposed basement membrane extracellular matrix, leukocytes, neutrophils monocytes, tumor cells, and other platelets. The same α-granules also release prothrombin, fibrinogen, factor V, and factor VIII that stimulate and promote coagulation and fibrin formation (56–66).
Platelet Activation and Liver Metastasis
The liver is a common site for cancer metastasis from primary tumors originating in the gastrointestinal tract. Traditionally the preponderance of liver metastasis was felt to be a function of drainage from the intestines into the hepatic circulation; however, the liver may instead represent a favorable microenvironment for metastasis. The liver’s unique regeneration may likewise contribute to creating a receptive metastasis-formation microenvironment involving platelets and the chronic activation of pathways related to the Myc family of regulator genes (287–292). More than half of CRC patients develop liver metastases (associated with poor prognosis) (85, 225) and an increased risk of thrombosis (241, 293). A positive correlation has been observed between high platelet counts and CRC tumor invasiveness and -metastasis, and a negative correlation between high platelet counts and survival in CRC patients (294–296). Platelet hyperactivation measured by elevated platelet mean volume or aggregation (297–301) can be a predictor of cancer progression in CRC (302–304). Genes implicated in CRC are also involved in platelet activation and coagulation suggesting a prothrombotic environment in CRC (305). During cancer progression and metastasis, platelet responses can be modulated by the tumor cells. Circulating tumor cells (CTCs) can stimulate heterotypic tumor-cell-induced platelet aggregation (TCIPA) (29, 85, 306). Tumor cells may get trapped within a TCIPA, go undetected by immune surveillance, be protected from shear forces while in circulation, and have greater capacity to migrate and metastasize (61, 271). Recently, a microfluidic approach was developed to isolate CTCs by targeting platelets that satellite on the tumor-cell surfaces. A significant number of platelet-coated CTCs was observed in metastatic cancer patients of both epithelial (lung and breast)- and non-epithelial (melanoma) tumor origins. Also observed were single cells and clusters, along with CTCs associated with leukocytes (307). Isolating CTCs using platelet markers emphasizes the potential of platelet-coated CTCs that go unnoticed by conventional isolation methods and their possible significance in metastasis.
Tumor cells also release thrombin which serves to activate platelets and results in the formation of tumor thrombi, a form of TCIPA. These thrombi could provide a supportive environment for CTCs by becoming tethered to blood vessels within distant tissues. Given the heightened coagulatory imbalances in the liver driven by cancer or pre-existing- and/or chemotherapy-induced chronic liver disease, these thrombi can become lodged within the liver sinusoids, potentially leading to the initiation of metastasis. Many studies have used anti-platelet drugs with chemotherapy to reduce platelet-mediated tumor-cell survival and metastasis. Platelets were also shown to promote angiogenesis by releasing angiogenic growth factors such as VEGF (308). In a study that provided aspirin along with tamoxifen therapy to patients with breast cancer investigated the impact of aspirin therapy on circulating levels of the proangiogenic-protein VEGF, the antiangiogenic-protein TSP‐1, and platelet-mediated angiogenic-protein release. The aspirin therapy not only impacted angiogenic protein levels but it may modify the angiogenic balance in women treated with tamoxifen therapy. The increase in antiangiogenic TSP‐1 levels without a concurrent increase in pro‐angiogenic VEGF levels suggests an anti-angiogenic balance from aspirin therapy. This study also found less release of platelet angiogenic proteins (309).
In addition to the pathways discussed previously, dysregulated modulation of TGFβ activity can also provide a favorable environment for tumorigenesis (76). Platelets are the major source of latent TGFβ which is released during platelet activation. This process also releases a furin-like proprotein convertase from platelets which in turn activates TGFβ. This enzyme-mediated activation was shown to continue in the damaged area even after platelet-derived TGFβ activation was complete (75). Aberrant expression of TGFβ could lead to liver fibrosis. Elevated levels of vWF can increase platelet binding to collagen in the cirrhotic condition (263). Thus, conditions like liver cirrhosis can increase the activation and aggregation of platelets, with TGFβ and other growth-factor release not only causing platelet binding with collagen, but also providing a tumorigenic niche within these platelet-collagen traps to capture CTCs and promote their growth within the liver.
Stake.com announced as principal sponsor of the European Cricket Championships
Stake.com has been announced as a principal partner of the European Cricket Championships, which will …
GiG seeks continued North American momentum as new hub opened
September 23, 2022
Platelet-Derived Serum Contents
The primary serum constituents resulting from platelet-initiated coagulation, growth, and wound repair are derived from intracellular sources (43–47). Circulating, resting platelets contain multiple storage granules: 1) alpha-granules (α-granules) 2) dense granules (δ-granules) 3) lysosomes, and 4) microparticles (48–50). There is also a newly described T-granule that primarily contains toll-like receptors 9 (51).
Liver Fibrosis
Liver disease is often associated with a marked decrease in the synthesis of proteins involved in coagulation. Whereas the levels of some factors like factor VIII, fibrinogen, and vWF remain unchanged or even increase due to defects in their clearance mechanism. In most cases, the defects in liver function are stabilized by between-coagulant- and anti-coagulant-protein counterbalancing. However, irreparable liver damage caused by conditions such as viral hepatitis and fatty liver can result in fibrosis of the liver. Some coagulation proteins—especially the prothrombotic factors—initiate microthrombi formation which could accelerate the progression of fibrosis. Studies in animal models have shown that factor Xa and thrombin contribute to liver fibrosis by occluding hepatic sinusoids with fibrin deposits (thus activating signaling pathways that promote a pro-fibrinogenic phenotype of the liver cells) or by triggering inflammation (156). Liver fibrosis, when severe, can progress into liver cancer and/or other life-threatening conditions. Patients with liver cirrhosis have an increased risk of all-cause mortality due to conditions like increased bleeding and thrombosis that can lead to severe, acutely life-threatening events like pulmonary thromboembolism. Budd-Chiari syndrome, which involves thrombotic occlusion and metastatic-tumor invasion of the hepatic veins, can also cause elevated portal pressures and ascites (210–212). Venous thromboembolism becomes particularly challenging in these patients (213). In others, conditions like liver cirrhosis may lead to a spectrum of coagulation defects, and in rare cases could even progress to disseminated intravascular coagulation—a common feature of end-stage liver disease—with widespread activation of coagulation, fibrinolysis, and hepatic failure. End-stage liver disease is also associated with thrombocytopenia and platelet dysfunction (214).
Liver Cell and Organ Biology
The normal liver exhibits unique heterogeneous and multifunctional organ properties, particularly at the cellular level (Figures 1–5A). Normal liver lobes are formed by parenchymal hepatocytes and non-parenchymal cells. Hepatocytes occupy nearly 80% of the total liver volume, play a role in innate immunity, and are responsible for much of the liver’s homeostatic and hemostatic functions using platelets widely distributed throughout the liver (Figures 1–4) (155, 156). Non-parenchymal cells represent 6.5% of the other liver-cell types that are organized around sinusoids.(Figure 4) The hepatic sinusoid walls are lined by sinusoidal endothelial cells (SECs), Kupffer cells, and HSCs along with intrahepatic lymphocytes, pit cells, and liver-specific, natural-killer cells (Figures 2–4) (157–167). Liver fibroblasts and myofibroblasts are thought to arise from multiple sources (168). Cholangiocytes and cells of the bile-canalicular system tend to associate preferentially with the hepatic arterial system (169).
FIGURE 1
Figure 1 Platelets in Hepatic Sinusoids and Vessels in the Normal Liver. Large numbers of platelets immunoreactive for CD61 in the hepatic sinusoids and vessels of the normal murine liver (20x).
FIGURE 2
Figure 2 Comparison of the Immune Composition of the Microenvironment in the Colon and Liver. Multiplex immunofluorescent images showing the immune composition of the human (A) and murine (B) colon (20x). Large numbers of IBA-1+ macrophages (red), CD3+ T cells (gold), and CD20+ B cells (green). Immune cells reside in the lamina propria of the mucosa and Peyer’s patches. In comparison, the microenvironment of a healthy liver has far fewer CD3+ T cells (gold) and CD20+ B cells (green), and is instead predominated by specialized macrophages termed Kupffer cells (red) (C). Insets show digitally magnified IBA-1+ macrophages (red), CD3+ T cells (gold) and CD20+ B cells (green) (not to scale). Image panel (D) shows both CD4+(orange) and CD8+(teal) T cells in the liver (insets are not to scale).
FIGURE 3
Figure 3 Resident and Bone Marrow-Derived Macrophages in the Liver. Multiplex immunofluorescent images of a normal murine liver showing the predominance of resident F4/80+ macrophages (red) with fewer bone marrow-derived CD11b+ macrophages (gold). Images also show S1008A expression (teal) by bone marrow-derived macrophages, but not resident macrophages. S1008A is involved in modulation of the immune response through cytokine secretion recruitment of leukocytes. Resident F4/80 macrophages also express arginase-1 consistent with M2, anti-inflammatory phenotype. Arginase-1 is widely expressed by hepatocytes.
FIGURE 4
Figure 4 Stromal Composition of the Liver and PDGRα Expression by Hepatic Portal Vessels. (A) Multiplex immunofluorescent images of a normal murine liver showing the predominance of collagen type I (red) around portal areas and collagen type IV (gold) lining hepatic sinusoids. (B) Image showing the co-localization of PDGFRα (green) with α-smooth muscle actin positive portal veins and hepatic arterioles (red) in the portal area of the liver.
FIGURE 5
Figure 5 (A) The normal liver sinusoid exhibits unique heterogeneous multifunctional cells. Hepatocytes (yellow, H) occupy the bulk of the liver volume. Non-parenchymal liver cells represented are primarily organized around sinusoids. Liver sinusoidal endothelial cells (LSEC) line the walls of the sinus and have open-pore capillaries with 100-300 nm fenestrations (F). Resting Ito cells contain lipocytes or vitamin A and fat-storing vesicles (yellow droplets) are hepatic stellate cells (HSC). Resident immune cells consist of Kupfer cells (K), intrahepatic lymphocytes (IHL), pit cells (PC), or liver-specific natural-killer (LSNK) cells. Liver fibroblasts and myofibroblasts are thought to arise from multiple mesenchymal sources. Cholangiocytes and cells of the bile-canalicular system tend to associate preferentially ductal junctions that converge at the canals of Hering. The space of Disse surrounds the sinusoids and constitutes a stem-cell niche that harbors HSCs or liver-resident mesenchymal stem cells (MSCs) that patrol and regulate space function. HSCs or antler stem cells (ASC), MSCs and other cells freely migrate within the space of Disse and contribute to regeneration, liver fibrosis, carcinogenesis, and metastasis. HSC in the perisinusoidal space of Disse are characterized by the presence of well-branched cytoplasmic processes which contact endothelial cells. Circulating tumor cells (CTC) can stimulate resting platelets (RP) to become activated (AP) and release their stored granule contents. (B) Multiple studies (first reported by Dingemans) have shown that platelets respond within minutes of encountering platelets in the liver sinusoids (153, 154). Five minutes after injection, tumor cells had formed large emboli that were present in portal branches. Tumor emboli (TC) adhered to the vascular-wall liver sinusoidal endothelial cells (LSEC) without fully occluding the lumen. On the vascular-lumen side of the emboli, aggregated and degranulated platelets were common with leukocyte and neutrophil associations whereas erythrocytes were found near the non-platelet-involved tumor-cell surfaces. Degranulated platelets an outer zone of the platelet aggregates with a closely packed, activated-platelet inner zone containing fibrin deposits. Although not shown, in other studies Kupffer cells were engaged and engulfed by tumor cells.
Liver sinusoids, by contrast, are lined by discontinuous liver sinusoidal endothelial cells (LSECs) open-pore capillaries with 50-150 nm gaps on average that can also occur less frequently as 200-300 nm gaps as measured by SEM and atomic force microscopy (166, 167, 170, 171). These low-pressure sinusoids provide a porous separation from the underlying parenchyma, and thus play an essential role in maintaining metabolic and immune homeostasis while they actively contribute to disease pathophysiology (172). LSECs exhibit endocytic and scavenging functions and facilitate nutrient exchange, which contributes to receptor-mediated clearance of waste products via scavenger receptors (SR-B1, SCARF1) and immunoglobulin-G complexes (CD32b). Capillarization and dysfunction precede fibrogenesis. LSECs, along with other microenvironmental components, play an active role in liver disease (173). LSECs stretch receptors in particular can sense deformation and wall shear that leads to CXCL1 release via integrin-dependent activation of transcription factors regulated by Notch proteins and their interaction with the mechano-sensitive Piezo calcium channel (174). This results in the recruitment of neutrophils, and the generation of sinusoidal microthombi that can promote portal hypertension (174). Platelet-LSEC interactions may involve P-selectin expression (175). These platelet-LSEC interactions can also promote immune tolerance and recruit distinct immunosuppressive leukocyte subsets that allow persistence of chronic viral infections and facilitate tumor development (173). LSECs are also thought to modulate the tumor microenvironment and promote development of a pre-metastatic niche, which can drive formation of secondary liver tumors (176).
Liver sinusoids receive blood from the hepatic artery and portal vein that empty into central veins. The space of Disse surrounds the sinusoids and constitutes a stem-cell niche that harbors HSCs or liver-resident mesenchymal stem cells that patrol and regulate cellular function within this microenvironment (177–181). HSCs, antler stem cells, mesenchymal stem cells, and other cells freely migrate within the space of Disse and contribute to regeneration, liver fibrosis, carcinogenesis, and metastasis (177–185). The complexities of this resident stem-cell space may contribute to the liver becoming an organ of extra-medullary hematopoiesis (Figure 5A) that is also prone to tumor metastasis.
Among the many mediators involved in the intercellular communication in the liver, some include prostanoids, nitric oxide, endothelin-1, TNF-alpha, interleukins, chemokines, growth factors (TGF-beta, PDGF, IGF-I, hepatocyte growth factor), reactive oxygen species, and vitamin A.
LSECs perform key filtration functions due to small diaphragm-windowed fenestrae or sieve plates that allow free diffusion of many substances between the blood and the hepatocyte surface. LSECs exhibit endocytic capacity for glycoproteins, components of the extracellular matrix (such as hyaluronate, collagen fragments, fibronectin, or chondroitin sulphate proteoglycan), transferrin, ceruloplasmin, and immune complexes. LSECs may also serve as MHC-I- and MHC-II-presenting cells involved in antigen-specific T-cell tolerance. They are also active in the secretion of eicosanoids, nitric oxide, cytokines, endothelin-1, and some extracellular-matrix components.
HSCs in the peri-sinusoidal space of Disse are characterized by the presence of well-branched cytoplasmic processes which contact endothelial cells. In the normal liver, HSCs (i.e. Ito cells) are able to store fat droplets and vitamin A. HSCs also control extracellular matrix turnover and regulate the contractility of sinusoids. Acute damage to hepatocytes activates transformation of quiescent stellate cells into myofibroblast-like cells that play a key role in the development of inflammatory fibrotic response (177–181). HSCs can also swell or contract due to the presence of smooth-muscle actin to regulate sinusoidal blood flow (186). Constriction or relaxation of HSCs from circulating molecules released by neighboring hepatocytes (carbon monoxide and leukotrienes), endothelial cells (endothelin, nitric oxide, and prostaglandins), Kupffer cells (prostaglandins and nitric oxide), and other stellate cells (endothelin and nitric oxide) (187).
Kupffer cells—intra-sinusoidal endocytic and phagocytic tissue macrophages that reside in the liver—are continuously exposed to gut-derived particulate materials and soluble bacterial products. They can produce reactive-oxygen species, nitric oxide, carbon monoxide, eicosanoids, TNF-α, and other cytokines as part of their innate immune-defense function. Kupffer cells can release these inflammatory mediators and ultimately damage the liver during liver dysfunction or when overexposed to bacterial products. Kupffer cells also engulf and clear senescent and damaged erythrocytes and platelets. In the cancer-research setting, Kupffer cells engulf tumor cells very early during metastasis following the injection of tumor cells into the portal vein (188). Liver macrophages modulate immune responses via antigen presentation and suppression of T-cell activation by antigen-presenting sinusoidal endothelial cells via paracrine actions of IL-10, prostanoids, and TNF-alpha. Kupffer cells can also secrete enzymes and cytokines during liver injury and inflammation that damage hepatocytes and remodel liver tissues.
Pit cells—liver-associated large granular lymphocytes like natural-killer cells—kill a variety of tumor cells in a major histocompatibility complex, unrestricted way, and this anti-tumor activity may γδ T cells, both conventional- and unconventional α- and β-T cells, or liver-sinusoidal natural-killer cells.
Single-cell sequencing technology has revealed more granular levels of cellular heterogeneity (6). These studies identified previously unknown subtypes of endothelial cells, Kupffer cells, and hepatocytes, associated with specific zonal population distributions (see data link: http://human-liver-cell-atlas.ie-freiburg.mpg.de). A zonal division of labor appears to exist within the organ (189, 190). Normal liver-lobule, metabolic, sub-specialization has been divided into the periportal zone that surrounds the portal triad (portal vein, hepatic artery, and bile duct), the central zone closest to the central vein, and the remaining mid zone (189–192). Single-cell sequencing of human liver has also uncovered distinct macrophage populations in those locations (193).
Platelet Activation and Aggregation
Trousseau first reported excessive blood coagulation in cancer patients with elevated platelet counts or thrombocytosis in 1865 (252–254). Since then, numerous studies have reported on thrombocytosis in cancer. In ovarian cancer, thrombocytosis is linked to elevated tumor interleukin-6 and liver-generated thrombopoietin and is associated with shortened overall survival of patients (255). In one study, orthotopic ovarian-mouse models revealed tumor-derived, interleukin-6-stimulated, hepatic-thrombopoietin (TPO) synthesis and paraneoplastic induction of thrombocytosis (255). Liver metastasis along with Fusobacterium in the liver can also trigger the thrombotic Trousseau’s syndrome (256–258). Observed abnormalities associated with coagulation factors like fibrinogen and prothrombin in liver disease could activate platelets (259). In certain conditions, platelets interact and bind with endothelial cells, hepatocytes, hematopoietic stem cells, and Kupffer cells in the liver (173). Upon injury to the liver endothelium, platelets are sequestered within the sinusoid and lead to endothelial-cell activation. This activation releases cytokines that facilitate the infiltration of immune cells (175). Recruitment of neutrophils and macrophages by platelets is of importance in liver disease as they regulate inflammation, fibrinogenesis, and fibrinolysis, and contribute to fibrosis (260). Platelet-mediated neutrophil recruitment and the interaction of platelets with the vasculature have been suggested as mechanisms driving cholestasis-induced liver damage (261). In NAFLD, the severity of inflammation and fibrosis in the liver was shown to directly correlate to the increased platelet turnover (262). In cirrhosis, the elevated levels of vWF promote platelet binding to collagen (263). Damage to the liver can directly affect liver-produced TPO levels. Circulating TPO levels have been shown to negatively correlate with the stage of liver disease, while reduced levels can lead to decreased platelet production or thrombocytopenia. In addition, thrombocytopenia in chronic liver disease is also caused by hypersplenism and increased sequestration of splenic platelets and increased platelet destruction due to aggregation in the liver (264). Increased thrombosis in these patients may consume platelets leading to lower levels in circulation (265). PDGF‐β, a mediator of hepatic fibrosis, is produced by platelets and released upon their activation (266). With the established association between platelet activation and liver fibrosis, anti-platelet drug use could prove beneficial in combating platelet-mediated liver disease and progression to cirrhosis. Recently, a systemic review and meta-analysis report of 3,141 patients concluded that the use of anti-platelet agents (such as aspirin or clopidogrel) was associated with a 32% decreased odds of hepatic fibrosis (adjusted pooled OR 0.68; CI 0.56–0.82, p ≤ 0.0001) (267). However, a prospective cohort study of patients at high risk of cardiovascular events revealed an inverse association between the use of anti-platelet agents and the presence and degree of liver fibrosis (268). A recent study of patients with hepatocellular carcinoma demonstrated that anti-platelet therapy was associated with improvement in overall survival and reduction in liver-related deaths. This study also reported that the use of anti-platelet therapy tended to delay the deterioration of liver function in these patients (269). Recent summaries of outcomes data in cancer prevention have also highlighted the cancer mitigating role of aspirin and other agents (270).
Therapy-Induced
Damage to the liver and hepatotoxicity can result from chemotherapy. This chemotherapy-induced injury is idiosyncratic and can range from asymptomatic, reversible, functional defects to advanced-stage liver disease like cirrhosis. Multiple factors like reactive-oxygen radicals, mitochondrial dysfunction, and immune dysregulation could affect the extent and degree of hepatotoxicity depending on the type of treatment received. Additionally, pre-existing liver conditions including nonalcoholic steatohepatitis are often aggravated by chemotherapy (215–217). Increased levels of activated platelets can also be identified in association with alcohol-induced liver cirrhosis and nonalcoholic steatohepatitis (218–220). Patients with hepatic sinusoidal-obstruction syndrome—associated with oxaliplatin-based chemotherapy (221)–experience portal hypertension, elevated liver enzymes, and splenomegaly, all of which can result in liver atrophy and fibrosis. Development of sinusoidal-obstruction syndrome in patients with CRC-based liver metastasis prior to liver resection surgery can lead to increased morbidity (221, 222). Increased incidence of liver damage is also emerging in association with checkpoint-blockade immunotherapy (223).
Cancer is a hypercoagulable state. Cancer-associated thrombosis increases the risk of morbidity and mortality in cancer patients (224). The risk of venous thromboembolism is approximately 4-fold higher in cancer patients than in normal individuals. Cancer-associated thrombosis also correlates with metastasis. Cancer cells can activate the coagulation cascade via signaling mechanisms and secretion of cytokines. Moreover, tumor burden can cause vessel compression eventually leading to thrombosis (224). Platelets are primary contributors in coagulation and thrombogenesis pathways. Platelet counts and activation markers have a significant impact on the prognosis of cancer and response to therapy (4, 225, 226). Intriguingly, cancer treatment, including surgery, also has an impact on platelet activity, which could increase hypercoagulability in these patients. In one study of cancer patients receiving chemotherapy, the risk of thrombosis was increased by 6- to 7-fold (227). Chemotherapy may also contribute to an increased risk of thrombotic events and venous thromboembolism, both associated with decreased survival. In another study, oxaliplatin-based chemotherapy affected platelets in the liver; the number attached to liver cells positively correlated with sinusoidal-obstruction syndrome severity (221). Oxaliplatin-based damage to hepatic sinusoids can possibly attract and activate platelets (228–230). Once activated, platelets secrete growth factors such as platelet-activating factor and thromboxane A2, causing liver injury, vascular and sinusoidal occlusion, and collagen deposition (231, 232). Then again, high-dose bevacizumab may interfere with platelet activation (228–230). The increased risk of bleeding in patients receiving bevacizumab treatment could be attributed to down-regulation of platelet activation (229). The impact of systemic cancer therapy is likely to vary along with the patient’s cancer type, performance status, comorbidities, individual platelet biology, and type of treatment (233–238).
Jdigital calls for open dialogue on Spanish gambling advertising laws
Jdigital – Spain’s online gambling trade association – has praised the decision of the Third …
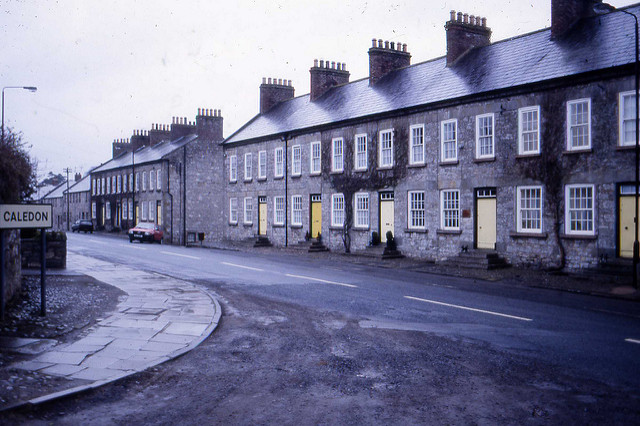
The UK government is regularly recognised for being a global leader in making public data openly available. Ministers have committed to being the most transparent government ever. We are determined to make sure that we keep producing high quality data and that we make it as accessible as possible.
What the Budget said
Last week’s Budget contained the following commitment:
The government will provide up to £5 million to develop options for an authoritative address register that is open and freely available. Making wider use of more precise address data and ensuring it is frequently updated will unlock opportunities for innovation.
Platelets and Minimal Residual Disease
Minimal residual disease (MRD)—a collection of viable cancer cells that are undetectable by standard imaging methods—differs by tumor type and requires disease-specific stratification based on distinctive organ-related microenvironmental disease characteristics (310). Recent discoveries in the development and use of liquid biopsies based on analyses of biomarkers in body fluids—including blood, urine, and cerebrospinal fluid—are showing potential for use in stratifying MRD. Liquid biopsy analyses can include (1) tumor-derived DNA, -RNA, -miRNA; epigenetic alterations; and proteins present in cell-free plasma or (2) contained in CTCs or (3) circulating exosomes and microvesicles or tumor educated platelets (271, 285, 286, 311–314). To fully understand MRD from a liquid biopsy standpoint, the inclusion of proteomics and metabolomics may be desirable to form a complete integrated molecular profile. To help with this, animal models of MRD involving gut related vascular modes of injection are materializing, which can help represent MRD in CRC (315, 316). Exposing platelets to tissue-factor-expressing tumor cells in the MRD microenvironment, may also trigger the activation cascade that promotes disease progression.
Based on our experience in developing in-house assays for solid tumor ctDNA (317), the detection of blood-based circulating-tumor DNA (ctDNA), a prognostic biomarker highly sensitive for CRC recurrence following curative-intent therapy, identifies MRD that will inevitably develop into clinically detectable, local recurrent- and/or distant metastases (318–321). Currently, ctDNA assays that detect MRD are also commercially available as a standard-of-care tool that enables oncologists to monitor for CRC recurrence. However, when ctDNA is detected, no prospective data directly linked to proven therapies are available to guide clinical management of CRC (322). A clinical need exists to better understand the biology of micrometastases and the role of platelets, especially in microscopic disease. If platelet-based biomarkers (e.g., platelet-to-lymphocyte ratios or micrometastasis-educated platelets) can portray ctDNA-defined MRD before the onslaught of macroscopic, clinically evident metastatic disease, our diagnosis and window for treating microscopic disease will likely improve before the tumor growth outpaces the immune-system ability to eliminate the disease. Presently, little is known from patient samples about the impact of platelets on the biology of micrometastases, while few therapeutics that consider this potential platelet involvement have been tested in patients.
Check Also
Strafe.com to offer esports insights to an ‘eagerly-awaiting’ Dutch crowd
September 23, 2022
Related Articles
Liver Disease and Cancer Risk
Liver disease contributes to an increased risk of CRC through the gut-liver axis (239). As the liver plays important roles in metabolism, synthesis and regulation of hormones, microbiome factor clearance, and blood detoxification, any major alterations in the liver function can lead to clinical findings including hypertension, diabetes, and hyperlipidemia. Systemic alterations stemming from liver dysfunction also can lead to dysregulated cytokine production and immune-cell function that initiate malignant transformation of cells and/or promote survival of cancer cells in the liver. Fatty liver is a known risk factor for CRC and cirrhosis patients have nearly double the risk of developing the CRC (240). CRC is known to be associated with thrombocytosis, hypercoagulation, and thromboembolic events (241, 242). About 35-55% of CRC patients develop liver metastasis, and surgical resection of the metastasis can be curative in some of these patients (243). In most cases, systemic chemotherapy is used prior to and after resection. This becomes challenging in patients with pre-existing- or with chemotherapy-induced liver disease as the goal of liver-resection surgery is to preserve liver functionality by removing metastasis. Patients with liver cirrhosis have a higher mortality rate following CRC surgery (244).
As the liver is actively involved in the synthesis and regulation of hormones, chronic hepatocellular damage can lead to hormonal imbalance. Estrogen levels are elevated in men with alcoholic liver disease and in others with a high body-mass index. Liver cirrhosis in men increases their risk for breast cancer possibly due to hyperestrogenemia (245–247). Steroid imbalance is also associated with viral cirrhosis and hepatocellular carcinoma (248). Evidence suggests that irrespective of cirrhosis type, all patients with cirrhosis and any related metabolic changes have an increased risk for liver cancer (249, 250). Studies also show that NAFLD is positively correlated with pancreatic cancer, and that NAFLD may serve as a prognostic factor; patients with pancreatic cancer and NAFLD have poorer overall survival than those without NAFLD (251). In addition, coagulation-factor Xa was shown to promote tumor growth and metastasis in animal models of melanoma (166).
Tumor Metastasis of the Liver
Ultrastructural studies of experimental metastasis to the liver were first reported by Dingemans (153, 154) (Figure 5B), who injected mammary carcinoma cells into syngeneic C57/Bl6 mice. Almost immediately after mesenteric-vein injection, tumor cells had formed large emboli in the portal branches. Tumor emboli adhered to the vascular wall without completely occluding the lumen. On the side of the embolus facing the vascular lumen, aggregated platelet clusters were found as part of the first response in the liver. On the luminal side of the platelet clusters, leukocytes, especially neutrophils, had adhered. Erythrocytes, by contrast, were present near the non-platelet-involved tumor cell surfaces, potentially suggesting that immune cells were preferentially attracted to the aggregated platelets. An outer zone consisting of the platelet aggregates was formed by degranulated- and more or less spherical platelets. The platelet aggregate centers consisted of closely packed, elongated elements along with small amounts of fibrin within heterogenous emboli, which were gradually displaced during metastatic growth and disease progression.
Platelet Lysosomes
Lysosome function in platelet biology and hemostasis is not well characterized (50, 55, 90, 91, 98, 129–134). Because they release phospholipase A, protease, and glycohydrolase enzymes, this suggests that lysosomes play a role in platelet responses and dissolution of clots (129, 132–134). Successive waves of platelet activation, adhesion, aggregation, and stabilization allow these first responders to activate specific subsets of cytoskeletal changes to recognize and secure vascular lesions.
Tumor-Educated Platelets and Exosomes
Tumor-derived exosomes—vesicles secreted by tumor cells—are packed with proteins and nucleic acid content that differs among different tumor types (271). These cancer exosomes may prime the liver environment to influence metastasis and can influence diverse cell types ranging from immune cells, endothelial cells, and platelets. Tissue factor—involved in the initiation of clot formation—may be enriched in cancer exosomes which may trigger increased thrombosis (272). Studies have also shown that microvesicles can bind and fuse with activated platelets to initiate coagulation (273).
Platelets also release a variety of vesicles upon activation, ranging from smaller ectosomes to larger exosomes. Platelet microvesicles make up the bulk of circulating microparticles in the blood stream (271, 274–278). These vesicles carry mRNA, miRNA, proteins, and lipids and are known to be critical in the process of angiogenesis, cancer progression, and metastasis (279, 280). When cells in the vicinity of platelet activation come into contact with the cargo in these vesicles, gene-based modulation of protein expression in target cells may occur (281). These vesicles can fuse with tumor cells and transfer receptors inducing chemotaxis, expression of metalloproteases, and cell proliferation. These exchanges may contribute to the development of drug resistance in tumor cells (282, 283). Recent findings provide insight into horizontal-RNA transfer mechanisms between platelets, platelet microparticles, and tumor cells (271, 284). Tumor-educated platelets appear to undergo modifications when they come into contact with tumor cells. These platelets may retain tumor-specific information including the primary tumor location (285, 286).
Platelet-Dense δ-Granules
Dense-granules are derived from the platelet endosomal lineage compartment. Early endosomes are currently thought to mature in multivesicular bodies. Like melanosomes, biogenesis of lysosome-related organelles complex (BLOC1 and -2) are involved with cellular exit of tubular structures that transport cargo from the endosomes to maturing δ-granules (83). The adapter-protein complex-3 may also elicit biogenesis of lysosome-related organelle complexes potentially involving BLOC1 and BLOC2. δ-granules that are generated from BLOC-containing organelle complexes can number 3-8 per human platelet and primarily contain bioactive small molecules. These δ-granules are released into the bloodstream during degranulation to enhance platelet activation, adhesion, and stabilization at sites of vascular damage. A number of ions (including calcium, magnesium, phosphate, and pyrophosphate) are released into the developing lesion microenvironment and influence platelet aggregation, clot progression, and wound evolution. Platelet δ-granules also actively accumulate and sequester nucleotides ATP, GTP, ADP, GDP and cyclic nucleotides via multidrug-resistance protein 4 (i.e., ABCC4), a transport pump for cyclic nucleotides and nucleotide analogs (86, 87). The exposure of tetraspanins and lysosomal-associated membrane protein-2 in association with platelet activation also occurs during release of δ-granules (88–91). Platelet δ-granules also release transmitters like serotonin (5-HT), epinephrine, and histamine (92, 93), which influence vascular function, macrophages, thrombosis, liver regeneration, and cancer progression (70, 94–97). Both α- and δ-granule release amplify secondary platelet responses, initiate wound repair, and influence cancer-cell proliferation (55, 98–100).
Septic Injury
Similar mechanisms are involved in a septic insult to the liver (205, 206). Severe sepsis induces dysregulated inflammation and coagulation leading to multiple organ (particularly liver) failure. Platelet TLR4 receptors initiate the formation of NETs to ensnare pathogens (205, 206). These platelet-initiated NETs and the extravasated platelet aggregation facilitate detachment of LSECs and trigger sepsis-induced liver dysfunction (205, 206). In the case of CRCs, invasive microorganisms like the bacterial species Fusobacterium nucleatum are often present (207). These and other bacteria can cause platelet aggregation and have been associated with cancer cells in metastatic lesions (207–209).
Making geospatial data even more usable
Nearly everything that happens has a link to a physical location. Address data serves a broader purpose than the delivery of post, parcels and services. It anchors everything to a specific place, and it’s often this anchor that’s used to connect other types of data together. So for a modern economy, high-quality geospatial data, linked to the addresses that people use on a day to day basis, is incredibly important.
The Budget announcement is an exciting government commitment to explore how an open address register can enable innovation, meet user needs, and deliver substantial and tangible benefits to the public and the economy by enabling new products and services.
Conflict of Interest
AKS: Consulting (Merck, Kiyatec), shareholder (BioPath), research funding (M-Trap).
The remaining authors declare that the research was conducted in the absence of any commercial or financial relationships that could be construed as a potential conflict of interest.
Work ahead
GDS Data Group and the Department for Business, Innovation and Skills (BIS) are working in conjunction with a range of other stakeholders to explore how to fully exploit the benefits of open and freely available address data.
We know that we have a lot of work to do, and a lot of people to engage, as we move forward. We are aware of the fundamental role played by local authorities in the creation and maintenance of addresses, and the important contributions of Geoplace, Ordnance Survey and Royal Mail. We look forward to continuing these conversations over the coming months.
Join the conversation on Twitter, and don't forget to sign up for email alerts.
Spain’s DGOJ sanctions autumn clean-up of licensee remits
Ted Menmuir October 22, 2019Europe, Latest News, SportsbookComments Off on Spain’s DGOJ sanctions autumn clean-up of licensee remits
Share
- Stumbleupon
Four Spanish licensed incumbents have had specific licensee provisions withdrawn ‘for inactivity’ sanctioned by gambling regulatory authority the DGOJ.
This October, the DGOJ undertook a review of operators’ current market status, which forced the regulator to terminate specific licensee provision attached to Unidad Editorial Juegos, Beatya Entertainment, Paf Consulting ABP and Winamax Espana.
Publishing its judgement, the DGOJ details that it has withdrawn the horseracing wagering remits of ‘Editorial Juegos’ – the operating company of MarcaApuestas.com, the online sportsbook domain of leading Spanish sports newspaper Marca.
Meanwhile, Åland Islands operator Paf, who has operated in the Spanish online gambling market since 2012, has seen its online bingo B2C licence terminated for failure to activate its product.
Of notable surprise, the DGOJ has withdrawn the B2C sports betting licence of French online gambling incumbent Winamax, who will now serve the Spanish market solely as an online poker operator.
This summer, Winamax announced its first La Liga football partnerships with newly-promoted Granada CF (currently third in La Liga), in which the French operator detailed that it would advertise both its online poker and sports betting services.
Winamax maintains the majority of its Spanish market presence as lead online poker partner for Marca, organising the sports publishers ‘Marca Poker’ national tour.
DGOJ’s final sanction relates to Beatya Entertainment, operating subsidiary of online casino StarVegas.es which has seen its licensing provisions for Punto y Banca table games axed.
Share
- Stumbleupon
Tags Granada CF marca Marcaapuestas Spain starvegas.es Unidad Editorial Juegos Winamax
α-Granule Growth and Angiogenesis Factors
Infiltrating- and tissue-resident immune cells, stromal cells, and fibroblasts are stimulated by α-granule factors. Growth factors include platelet-derived growth factor (PDGF), basic fibroblast growth factor (48, 67), epidermal growth factor (EGF) (68), hepatocyte growth factor (69, 70), insulin-like growth factor 1 (71, 72), and transforming growth factor-beta (TGFβ) (73–79)—all of which can induce cell-type- and function-specific proliferation and immunomodulation. Pericytes, smooth muscle fibroblasts (myofibroblasts), and endothelial cells are stimulated by α-granule-released pro-angiogenic and anti-angiogenic factors that include vascular endothelial growth factors (VEGF)-A and -C) (80, 81), angiopoietin-1, angiostatin, and sphingosine-1-phosphate (48, 59, 67, 80, 82–85).
New articles
Gold Fish Casino Slots Free Coins, Redemption and Redeem Codes – Collect Game Coins, Free Chips, Spins, Bonus, Redeem Codes
Gold Fish Casino Slots Free Coins, Redemption and Redeem Codes – Collect Game Coins, Free Chips, Spins, Bonus, Redeem Codes
Download Royal Vegas Android App To Play Slots In Ireland
How to download Royal Vegas Android app to play slots In Ireland? Download the online casino app for Android or iPhone and play slots, table games.
Slots Casino Jackpot Ireland Billionaires Trust:Amazon.co.uk:Appstore for Android
Slots Casino Jackpot Ireland Billionaires Trust:Amazon.co.uk:Appstore for Android
Encore Classic Casino Games
Encore Classic Casino Games
Friends Mobile Slot Review | WMS
Friends slot gives you the chance to catch up with the gang, visit Central Perk, play with Wilds, Mystery Stacks & Jackpots. Find out more in our mobile review
Penny Slot Machine - Etsy Vietnam
Check out our penny slot machine selection for the very best in unique or custom, handmade pieces from our collectibles shops.
Online Slots| Genesis Casino
There’s a plethora of Online Slots available at Genesis Casino. You can choose from a wide range of themes from classic fruit games to adventurous games.